Anatomy and Physiology
Copyright ©
March 2003 Ted Nissen
Table
of Contents
1 Introduction. 2
2 Chemical Organization. 2
3 Cellular Organization. 28
4 Tissue Organization. 28
5 Integumentary System* 51
6 Skeletal Tissue. 52
7 Skeletal System-Axial* 52
8 Skeletal System-Appendicular Skeleton* 57
9 Articulations. 60
10 Muscle Tissue. 77
11 Muscular System* 88
12 Nervous Tissue* 88
13 Spinal Cord and Spinal Nerves* 91
14 The Brain and Cranial Nerves. 101
15 Sensory, Motor, and Integrative
Systems. 109
16 Autonomic Nervous System. 109
17 Special Senses. 109
18 Endocrine System* 109
19 Cardiovascular System-Blood* 111
20 Cardiovascular System-Heart 111
21 Cardiovascular System-Vessels and Routes. 112
22 Lymphatic and Immune System* 114
23 Respiratory System* 114
24 Digestive System* 114
25 Metabolism. 114
26 Urinary System* 114
27 Fluid, Electrolyte, and
Acid-Base Dynamics. 114
28 Reproductive System* 114
29 Development and Inheritance. 114
2.1 General Introduction
2.1.1 Many of the common substances we eat and drink-water, sugar, table
salt, cooking oil-play vital roles in keeping us alive. In this chapter, you
will learn something about how these substances function in your body.
Fundamental to this study is knowledge of basic chemistry and chemical
processes, since your body is composed of chemicals and all body activities are
chemical in nature. To understand the nature of the matter you are made from
and the changes this matter goes through in your body, you will need to know
which chemical elements are present in the human organism and how they
interact.
2.2 Introduction to Basic Chemistry
2.2.1 Chemical Elements
2.2.1.1
All living and nonliving things
consist of matter, which is anything that occupies space and has mass. Matter
may exist in a solid, liquid, or gaseous state. All forms of matter are made up
of a limited number of building units called chemical elements, substances that
cannot be decomposed into simpler substances by ordinary chemical reactions. At
present, scientists recognize 106 different elements, of which 92 occur
naturally. Elements are designated by letter abbreviations, usually derived
from the first or first and second letters of the Latin or English name for the
element. Such letter abbreviations are called chemical symbols. Examples of
chemical symbols are H (hydrogen), C (carbon), 0 (oxygen), N (nitrogen), Na
(sodium), K (potassium), Fe (iron), and Ca (calcium).
2.2.1.2
Periodic Table Web Reference
2.2.1.3
Approximately 26 elements are found in
the human organism. Oxygen, carbon, hydrogen, and nitrogen make up about 96
percent of the body's weight. These four elements together with calcium and
phosphorous constitute approximately 99 percent of the total body weight.
Twenty other chemical elements, called trace elements, are found in low
concentrations and compose the remaining 1 percent.
2.2.2 Particle Physics Link
2.2.3 Structure of Atoms
2.2.3.1
Each element is made up of units of matter
called atoms, the smallest units of matter that enter into chemical reactions.
An element is simply a quantity of matter composed of atoms all of the same
type. A handful of the element carbon, such as pure coal, contains only carbon
atoms. A tank of oxygen contains only oxygen atoms. Measurements indicate that
the smallest atoms are less than 0.00000001 cm 1/250,000,000 inch) in diameter,
and the largest atoms are 0.00000005 cm (1/50,000,000 inch) in diameter. In
other words, if 50 million of the largest atoms were placed end to end, they
would measure approximately 2.5 cm (1 inch) in length.
2.2.3.2
An atom consists of two basic parts:
the nucleus and electrons (Figure 2-1).
2.2.3.3
Illustration (Figure 2-1)
2.2.3.3.1 Introduction
2.2.3.3.1.1 Structure of an atom.
In this highly simplified version of a carbon atom, note the centrally located
nucleus. The nucleus contains six neutrons and six protons, although all are
not visible in this view since some are behind others. The six electrons move
about the nucleus at varying distances from its center
2.2.3.3.1.2 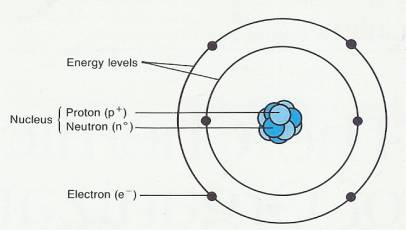
2.2.3.4
The centrally located nucleus
constitutes most of the atomic mass and contains positively charged particles
called protons (p+) and uncharged (neutral) particles called
neutrons (nO). Because each proton has one positive charge, the
nucleus itself is positively charged. Together, protons and neutrons are
referred to as particles called nucleons. Various coordinated movements of
nuclear particles cause the nucleus to vibrate in several distinctive patterns.
Electrons (e-) are negatively charged particles that move around the
nucleus. For reasons far beyond the scope of this text-. book, the diagrams of
atoms are very simplified, resembling planetary models of the solar system. (In
actuality, electrons do not follow fixed paths around the nucleus but move
about in probable locations.) The number of electrons in an atom of an element
always equals the number of protons. Since each electron carries one negative
charge, the negatively charged electrons and the positively charged protons
balance each other, and the atom is electrically neutral.
2.2.3.5
The standard relative weight unit for
measuring subatomic particles and atoms is called an atomic mass unit (amu) or
dalton. A neutron has a mass of 1.008 daltons, and a proton has a mass of 1.007
daltons. The mass of an electron is 0.0005 daltons, about 1/2000 the mass of a
neutron or proton.
2.2.3.6
What makes the atoms of one element
different from those of another? The answer lies in the number of protons.
Figure 2-2 shows that the hydrogen atom contains one proton; the helium atom
contains two; the carbon atom has six; and so on. Each different kind of atom
has a different number of protons in its nucleus. The number of protons in an
atom is called the atom's atomic number. Therefore, we can say that each kind
of atom, or element, has a different atomic number. The total number of protons
and neutrons in an atom is its approximate atomic weight. Each proton and
neutron contributes one dalton unit of weight to the atom. Thus, an atom of sodium
has an atomic weight of23 because of the presence of 11 protons and 12 neutrons
in its nucleus.
2.2.3.7
Illustration (Figure 2-2) Atomic
Structures of some Representative Atoms
2.2.3.7.1 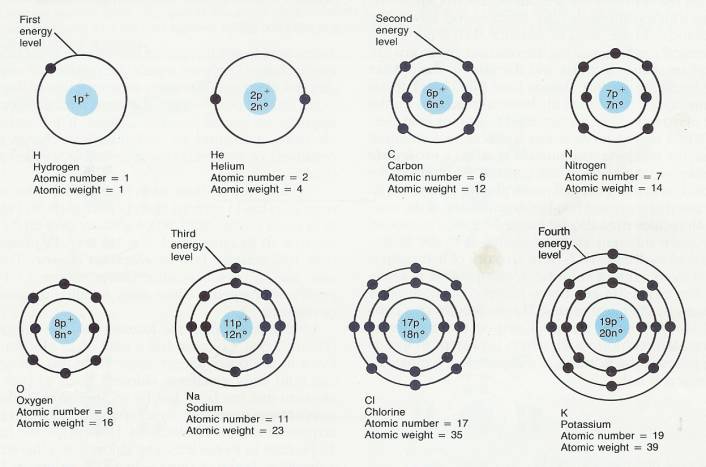
2.2.3.8
Atoms of an element, although
chemically alike, may have different nuclear masses and thus different atomic
weights because of one or more extra neutrons in some of the atoms, so the
atomic weight assigned to an element is only an average. Each of the chemically
identical atoms of an element with a particular nuclear mass is an isotope of
that element. All isotopes of an element have the same number of protons in
their nuclei, but their atomic weights differ because of the difference in the
number of neutrons. In a sample of oxygen, for example, most atoms have 8
neutrons, but a few have 9 or 10, even though all have 8 protons. The isotopes
of oxygen are designated as 160, 170, and 180.
The numbers indicate their atomic weights.
2.2.3.9
Certain isotopes called radioisotopes
are unstable they "decay" or change their nuclear structure to a more
stable configuration. And in decaying they emit high energy radiation (alpha,
beta, or gamma particles) that can be detected by instruments. These
instruments estimate the amount of radioisotope present in a part of the body
or in a sample of material and form an image of its distribution. (Refer to the
discussion of positron emission tomography, or PET, in Chapter 1.)
2.2.4 Atoms and Molecules
2.2.4.1
When atoms combine with or break apart
from other atoms, a chemical reaction occurs. In the process, new products with
different properties are formed. Chemical reactions are the foundation of all
life processes. .
2.2.4.2
The electrons of an atom actively
participate in chemical reactions. The electrons move around the nucleus in
regions, shown in Figure 2-2 as concentric circles lying at varying distances
from the nucleus. We call these regions energy levels. Each energy level has a
maximum number of electrons it can hold. For instance, the energy level nearest
the nucleus never holds more than two electrons, no matter what the element.
This energy level can be referred to as the first energy level. The second
energy level holds a maximum of eight electrons. The third level of atoms whose
atomic number. is less than 20 also can hold a maximum of eight electrons. The
third level of more complex atoms can hold a maximum of 18 electrons.
2.2.4.3
An atom always attempts to fill its
outermost energy level with the maximum number of electrons it can hold. To do
this, the atom may give up, take on, or share electrons with another
atom-whichever is easiest. The valence (combining capacity) is the number of
extra or deficient electrons in the outermost energy level. Take a look at the
chlorine atom. Its outermost energy level, which happens to be the third level,
has seven electrons. Since the third level of an atom can hold a maximum of
eight electrons, chlorine can be described as having a shortage of one
electron. In fact, chlorine usually does try to pick up an extra electron.
Sodium, by contrast, has only one electron in its outer level. This again happens
to be the third energy level. It is much easier for sodium to get rid of the
one electron than to fill the third level by taking on seven more electrons.
Atoms of a few elements, like helium, have completely filled outer energy
levels and do not need to gain or lose electrons. These are called inert
elements and are not chemically active.
2.2.4.4
Atoms with incompletely filled outer
energy levels, like sodium and chlorine, tend to combine with other atoms in a
chemical reaction. During the reaction, the atoms can trade off or share
electrons and thereby fill their outer energy levels. Atoms that already have
filled outer levels generally do not participate in chemical reactions for the
simple reason that they do not need to gain or lose electrons. When two or more
atoms combine in a chemical reaction, the resulting combination is called a
molecule (MOL-e-kyool). A molecule may contain two atoms of the same kind, as
in the hydrogen molecule: H2. The subscript 2 indicates that there
are two hydrogen atoms in the molecule. Molecules may also be formed by the
reaction of two or more different kinds of atoms, as in the hydrochloric acid
molecule: HCL. Here an atom of hydrogen is attached to an atom of chlorine. A
compound is a substance that can be broken down into two or more other
substances by chemical means. The molecules of a compound always contain atoms
of two or more different elements. Hydrochloric acid, which is present in the
digestive juices of the stomach, is a compound. A molecule of hydrogen is not.
2.2.4.5
The atoms in a molecule are held
together by electrical forces of attraction called chemical bonds, a form of
potential energy. As you will see, when chemical bonds are broken, energy is
released. When chemical bonds are formed, energy is required. Here we will
consider ionic bonds, covalent bonds, and hydrogen bonds.
2.2.4.6
CLINICAL APPLICADON: lasers
2.2.4.6.1 The
laser (acronym for light amplification by stimulated emission of radiation) is
based on a relatively simple principle. Atoms, molecules, or ions in a laser
are excited by absorption of energy (thermal, electrical, or optical). After
absorption, the atoms, molecules, or ions give off an equal amount of energy in
the form of light. This energy, the laser beam, is an intense light that can
produce surgical effects. These include coagulation to stop bleeding, incision
making, and tissue removal.
2.2.4.6.2 Clinical
applications have been found for lasers in several medical and surgical
specialties. Among these are ophthalmology (treatment of glaucoma, tumors,
cataracts), dermatology and plastic surgery (removal of lesions, port-wine
stains, and tattoos), cardiovascular surgery (angioplasty to open blood
vessels), gastrointestinal surgery (control of bleeding and tumor removal),
general surgery (incisions and removal of decubitus ulcers and leukoplakia),
gynecology (treatment of vulvar, vaginal, and cervical neoplasia and
endometriosis), neurosurgery (incision or removal of tissue near sensitive
neural and vascular structures), otolaryngology (tonsillectomy, treatment of
tumors of the larynx, and removal of nasal polyps), and urology (removal of
lesions and opening strictures).
2.2.4.7
Ionic Bonds
2.2.4.7.1 Atoms
are electrically neutral because the number of positively charged protons
equals the number of negatively charged electrons. But when an atom gains or
loses electrons, this balance is upset. If the atom gains electrons, it
acquires an overall negative charge. If the atom loses electrons, it acquires
an overall positive charge. Such a negatively or positively charged particle is
called an ion (I-on).
2.2.4.7.2 Consider
the sodium ion (Figure 2-3a). The sodium atom (Na) has 11 protons and 11
electrons, with 1 electron in its outer energy level. When sodium gives up the
single electron in its outer level, it is left with 11 protons and only 10
electrons. It is an electron donor. The atom now has an overall positive charge
of one (+ 1). This positively charged sodium atom is called a sodium ion
(written Na+).
2.2.4.7.3 Another
example is the formation of the chloride ion (Figure 2-3b). Chlorine has a
total of 17 electrons, 7 of them in the outer energy level. Since this energy
level can hold eight electrons, chlorine tends to pick up an electron that has
been lost by another atom. Chlorine is an electron acceptor. By accepting an
electron, chlorine acquires a total of 18 electrons. However, it still has only
17 protons in its nucleus. The chloride ion therefore has a negative charge of
one ( - 1) and is written as Cl-.
2.2.4.7.4 The
positively charged sodium ion (Na +) and the negatively charged chloride ion
(Cl-) attract each other-unlike charges attract each other. The attraction,
called an ionic bond, holds the two ions together, and a molecule is formed
(Figure 2-3c). The formation of this molecule, sodium chloride (NaCl), or table
salt, is one of the most common examples of ionic bonding. Thus, an ionic bond
is an attraction between ions formed when one atom loses electrons and another
atom gains electrons. Generally, atoms whose outer energy level is less than
half filled lose electrons and form positively charged ions called cations
(KAT-ī-ons). Examples of cations are potassium ion (K+), calcium ion
(Ca2+), iron ion (Fe2+), and sodium ion (Na +). By contrast, atoms whose outer
energy level is more than half-filled tend to gain electrons and form
negatively charged ions called anions (AN- ī -ons). Examples of anions
include iodide ion (I -), chloride ion (Cl-), and sulfur
ion (S2-).
2.2.4.7.5 Notice
that an ion is always symbolized by writing the chemical abbreviation followed
by the number of positive ( + ) or negative ( - ) charges the ion acquires.
2.2.4.7.6 Hydrogen
is an example of an atom whose outer level is exactly half-filled. The first
energy level can hold two electrons, but in hydrogen atoms, it contains only
one. Hydrogen may lose its electron and become a positive ion (H +). This is precisely
what happens when hydrogen combines with chlorine to form hydrochloric acid (H
+ Cl-). However, hydrogen is equally capable of forming another kind of bond
called a covalent bond.
2.2.4.8 Figure 2-3
2.2.4.8.1 Introduction
2.2.4.8.1.1 Formation of an ionic
bond. (a) An atom of sodium attains stability by passing a single electron to
an electron acceptor. The loss of this single electron results in the formation
of a sodium ion (Na+). (b) An atom of chlorine attains stability by accepting a
single electron from an electron donor. The gain of this single electron
results in the formation of a chloride ion (GI-). (c) When the Na + and the CI-
ions are combined, they are held together by the attraction of opposite
charges, which is known as an ionic bond, and a molecule of sodium chloride
(NaCI) is formed.
2.2.4.8.2 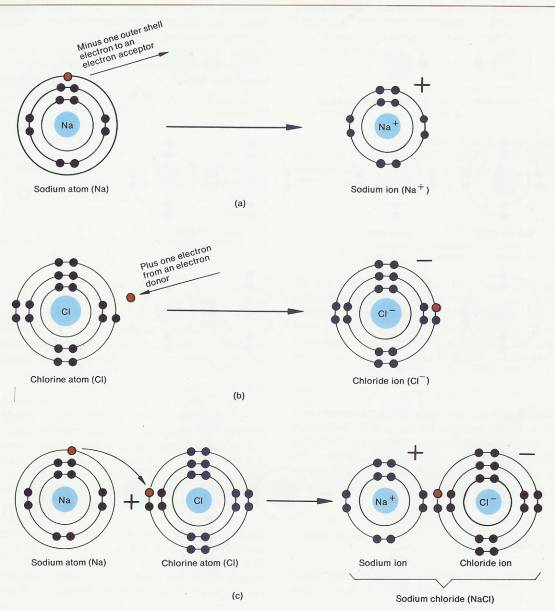
2.2.4.9 Covalent Bonds
2.2.4.9.1 The
second chemical bond to be considered is the covalent bond. This bond is far
more common in organisms than an ionic bond and is more stable. When a covalent
bond is formed, neither of the combining atoms loses or gains an electron.
Instead, the two atoms share one, two, or three electron pairs. Look at the
hydrogen atom again. One way a hydrogen atom can fill its outer energy level is
to combine with another hydrogen atom to form the molecule H2
(Figure 2-4a). In the H2 molecule, the two atoms share a pair of
electrons. Each hydrogen atom has its own electron plus one electron from the
other atom. The two electrons actually circle the nuclei of both atoms,
spending their time equally between both atoms. Therefore, the outer energy
levels of both atoms are filled half the time. When one pair of electrons is
shared between atoms, as in the H2 molecule, a single covalent bond
is formed. A single covalent bond is expressed as a single line between the
atoms (H-H). When two pairs of electrons are shared between two atoms, a double
covalent bond is formed, which is expressed as two parallel lines (=) (Figure
2-4b). A triple covalent bond, expressed by three parallel lines, occurs when
three pairs of electrons are shared (Figure 2-4c).
2.2.4.9.2 The
same principles that apply to covalent bonding between atoms of the same
element also apply to atoms of different elements. Methane (CH4),
also known as marsh gas, is an example of covalent bonding between atoms of
different elements (Figure 2-4d). The outer energy level of the carbon atom can
hold eight electrons but has only four of its own. Each hydrogen atom can hold
two electrons but has only one of its own. In the methane molecule the carbon
atom shares four pairs of electrons. One pair is shared with each hydrogen
atom. Each of the four carbon electrons orbits around both the carbon nucleus
and a hydrogen nucleus. Each hydrogen electron circles around its own nucleus
and the carbon nucleus.
2.2.4.9.3 FIGURE
2-4 Covalent Bond
2.2.4.9.3.1 Introduction
2.2.4.9.3.1.1 Formation
of a covalent bond between atoms of the same element and between atoms of
different elements. In the symbols on the right, each covalent bond is
represented by a straight line between atoms. (a) A single covalent bond between
two hydrogen atoms. (b) A double covalent bond between two oxygen atoms. (c) A
triple covalent bond between two nitrogen atoms. (d) Single covalent bonds
between a carbon atom and four hydrogen atoms.
2.2.4.9.3.2 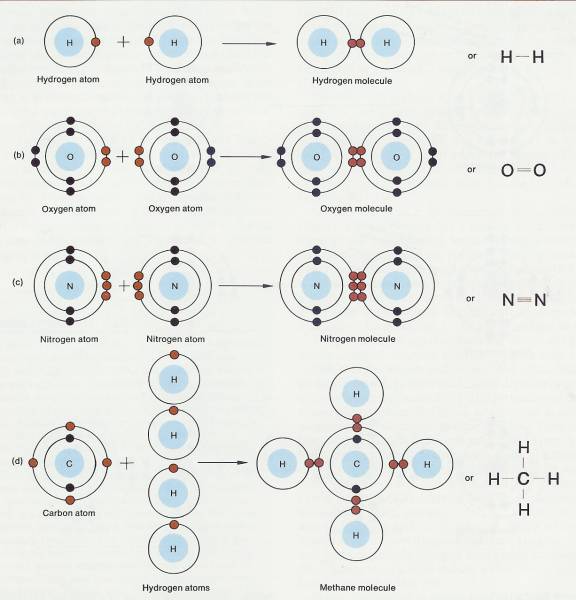
2.2.4.9.4 In
some covalent bonds, the electrons are shared equally between atoms, that is,
one atom does not attract the shared electrons more strongly than the other
atom. Such covalent bonds are referred to as nonpolar covalent bonds. Examples
include the bonds between hydrogen atoms and between oxygen atoms (see Figure
2-4a,b). In other covalent bonds, there is an unequal sharing of electrons
between atoms, that is, one atom attracts the shared electrons more strongly
than the other. This type of covalent bond is known as a polar covalent bond.
An example of this type of bond occurs in a molecule of water (see Figure 2-6).
This will be considered in more detail later in the chapter as part of the
discussion of the function of water as a solvent.
2.2.4.9.5 Elements
whose outer energy levels are half-filled, such as hydrogen and carbon, form
covalent bonds quite easily. In fact, carbon always forms covalent bonds. It
never becomes an ion. However, many atoms whose outer energy levels are more
than half-filled also form covalent bonds. An example is oxygen. We won't go
into the reasons why some atoms tend to form covalent bonds rather than ionic
bonds.
2.2.4.9.6 Hydrogen
Bonds
2.2.4.9.6.1 A hydrogen bond
consists of a hydrogen atom covalently bonded to one oxygen atom or one
nitrogen atom but attracted to another oxygen or nitrogen atom. Because hydrogen
bonds are weak, only about 5 percent as strong as covalent bonds, they do not
bind atoms into molecules. However, they do serve as bridges between different
molecules or between various parts of the same molecule. The weak bonds may be
formed and broken fairly easily. It is this property that accounts for the
temporary bonding between certain atoms within large complex molecules such as
proteins and nucleic acids (see Figure 2-12). It should be noted that even
though hydrogen bonds are relatively weak, such large molecules might contain
several hundred of these bonds, resulting in considerable strength and
stability.
2.2.4.9.6.2 Chemical reactions
are nothing more than the making, or breaking of bonds between atoms. And these
reactions occur continually in all the cells of your body. As you will see
again and again, chemical reactions are the processes by which body structures
are built and body functions carried out.
2.2.5 Chemical Reactions
2.2.5.1
As we said earlier, chemical reactions
involve the making or breaking of bonds between atoms. After a chemical
reaction, the total number of atoms remains the same, but because they are
rearranged, there are new molecules with new properties. In this section, we
will look at the basic chemical reactions common to all living cells. Once you
have learned them, you will be able to understand the chemical reactions
discussed later.
2.2.5.2
Synthesis Reactions-Anabolism.
2.2.5.2.1 When
two or more atoms, ions, or molecules combine to form new and larger molecules,
the process is called a synthesis reaction. The word synthesis means
"combination," and synthesis reactions involve the farming of new
bonds. Synthesis reactions can be expressed in the following way:
2.2.5.2.2 Illustration
Synthesis Reactions #1
2.2.5.2.2.1 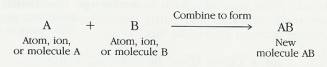
2.2.5.2.3 The
combining substances, A and B, are called the reactants; the substance formed
by the combination is the end product. The arrow indicates the direction in
which the reaction is proceeding. An example of a synthesis reaction is:
2.2.5.2.4 Illustration
Synthesis Reactions #2
2.2.5.2.4.1 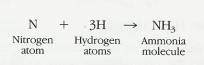
2.2.5.2.5 All
the synthesis reactions that occur in your body are collectively called
anabolic reactions, or simply anabolism (a-NAB-ō-lizm). Combining glucose
molecules to form glycogen and combining amino acids to form proteins are two
examples of anabolism. The importance of anabolism is considered in detail in
Chapter 25.
2.2.5.3
Decomposition Reactions-Catabolism
2.2.5.3.1 The
reverse of a synthesis reaction is a decomposition reaction. The word decompose
means to break down into smaller parts. In a decomposition reaction, the bonds
are broken. Large molecules are broken down into smaller molecules, ions, or
atoms. A decomposition reaction occurs in this way:
2.2.5.3.2 Illustration
Decomposition Reaction #1
2.2.5.3.2.1 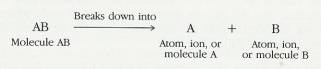
2.2.5.3.3 Under
the proper conditions, methane can decompose into carbon and hydrogen:
2.2.5.3.4 Illustration
Decomposition Reaction #2
2.2.5.3.4.1 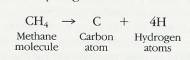
2.2.5.3.5 The subscript
4 on the left-hand side of the reaction equation indicates that four atoms of
hydrogen are bonded to one carbon atom in the methane molecule. The number 4 on
the right-hand side of the equation shows that four single hydrogen atoms have
been set free.
2.2.5.3.6 All
the decomposition reactions that occur in your body are collectively called
catabolic reactions, or simply catabolism (ka-TAB- ō -lizm). The digestion
and oxidation of food molecules are examples. The importance of catabolism is
also considered in detail in later Chapters.
2.2.5.4 Exchange Reactions
2.2.5.4.1 All
chemical reactions are based on synthesis or decomposition processes. In other
words, chemical reactions are simply the making and/or breaking of ionic or
covalent bonds. Many reactions, such as exchange reactions, are partly
synthesis and partly decomposition. An exchange reaction works like this:
2.2.5.4.1.1 Illustration
Exchange Reaction
2.2.5.4.1.1.1 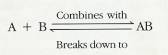
2.2.5.4.2 The
bonds between A and B and between C and D are broken in a decomposition
process. New bonds are then formed between A and D and between B and C or
between A and C and between B and D in a synthesis process.
2.2.5.5 Reversible Reactions
2.2.5.5.1 When
chemical reactions are reversible, the end product can revert to the original
combining molecules. A reversible reaction is indicated by two arrows:
2.2.5.5.2 Illustration
Reversible Reactions 1
2.2.5.5.2.1 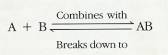
2.2.5.5.3 Some
reversible reactions reverse themselves only under special conditions:
2.2.5.5.4 Illustration
Reversible Reactions 2
2.2.5.5.4.1 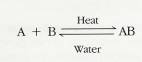
2.2.5.5.5 Whatever
is written above or below the arrows indicates the special condition under
which the reaction occurs. In this case, A and B react to produce AB only when
heat is applied, and AB breaks down into A and B only when water is added.
Figure 2-5 summarizes the basic chemical reactions that can occur.
2.2.5.6 How Chemical Reactions Occur
2.2.5.6.1 The
collision theory explains how chemical reactions occur and how certain factors
affect the rates of those reactions. According to this theory, all atoms, ions,
and molecules are continuously moving and colliding with one another. The
energy transferred by the particles in the collision might disrupt their
electron structures enough that chemical bonds are broken or new ones are
formed.
2.2.5.6.2 Several
factors determine whether a collision will actually cause a chemical reaction.
Among these are the velocities of the colliding particles, their energy, and
their specific chemical configurations. Up to a point, the higher the
particles' velocities, the greater the probability that their collision will
result in a reaction. Also, each chemical reaction requires a specific level of
energy. The collision energy required for a chemical reaction is its activation
energy, which is the amount of energy needed to disrupt the stable electronic
configuration of a specific molecule so that the electrons can be rearranged.
(The relationship of enzymes to activation energy is considered in Chapter 25.)
But even if colliding particles possess the minimum energy needed for reaction,
no reaction will take place unless the particles are properly oriented toward
each other.
2.2.5.7
Energy and Chemical Reactions
2.2.5.7.1 Energy
is the capacity to do work. The two principal kinds of energy are potential
(inactive or stored) and kinetic (energy of motion). Energy, whether potential
or kinetic, exists in a number of different forms.
2.2.5.7.2 Chemical
energy is the energy released or absorbed in the breaking or forming of
chemical bonds. When a chemical bond is formed, energy is required. Such a
chemical reaction is called an endergonic (energy inward) reaction. When a bond
is broken, energy is released. Such a chemical reaction is called an exergonic
(energy. outward) reaction. This means that synthesis reactions are endergonic
(need energy), whereas decomposition reactions are exergonic (give off energy).
The building processes of the body-the construction of bones, the growth of
hair and nails, the replacement of injured cells occur basically through
synthesis reactions. The breakdown of foods, on the other hand, occurs through
decomposition reactions. When foods are decomposed, they release energy that
can be used by the body for its building processes.
2.2.5.7.3 Radiant
energy, such as heat and light, travels in waves. Some of the energy released
during decomposition reactions is heat energy, which is used to help maintain
normal body temperature, but does not accomplish cellular work.
2.2.5.7.4 Electrical
energy is the result of the flow of charges, electrons, or charged particles
called ions. As you will see later, electrical energy is essential for the
conduction of action potentials by nerve and muscle cells.
2.2.5.7.5 The
various forms of energy can be transduced (transformed) from one form into
another. For example, potential energy can be transduced to kinetic energy and
kinetic energy can be transduced to potential energy.
2.3 Chemical Compounds and Life Processes
2.3.1 Most of the chemicals in the body exist in the form of compounds.
Biologists and chemists divide these compounds into two principal classes:
inorganic compounds and organic compounds. Inorganic compounds usually lack
carbon. They are usually small, ionically bonded molecules that are vital to
body functions. They include water and many salts, acids, and bases. Organic
compounds always contain carbon and hydrogen. Carbon is a unique element in the
chemistry of life. Because carbon has four electrons in its outer shell, it can
combine with a variety of atoms, including other carbon atoms, to form straight
or branched chains and rings. Carbon chains are. the backbone for many
substances of living cells. Organic compounds are held together mostly or
entirely by covalent bonds. Organic compounds present in the body include
carbohydrates, lipids, proteins, nucleic acids, and adenosine triphosphate
(ATP).
2.3.2 Figure 2-5
2.3.2.1
Description
2.3.2.1.1 FIGURE
2-5 Kinds of chemical reactions. (a) Synthetic or anabolic reaction. When
linked together as shown, molecules of glucose form a molecule of glycogen.
Glucose is a sugar that is the primary source of energy. Glycogen is a stored
form of that sugar found in the liver and skeletal muscles. (b) Decomposition
or catabolic reaction. The example shows a molecule of fat breaking down into
glycerol and fatty acids. This reaction occurs whenever a food that contains
fat is digested. (c) Exchange reaction. In this reaction, atoms of different
molecules are exchanged with each other. Shown is a buffer reaction in which
the body eliminates strong acids to help maintain homeostasis. (d) Reversible
reaction. ATP (adenosine triphosphate) is an important source of stored energy.
When such energy is needed, the ATP breaks down into ADP (adenosine diphosphate)
and PO43- (phosphate group), releasing energy in the
reaction. The phosphate group is symbolized as P. The cells of the body
reconstruct ATP by using the energy of foods to attach ADP to PO43-
2.3.2.2
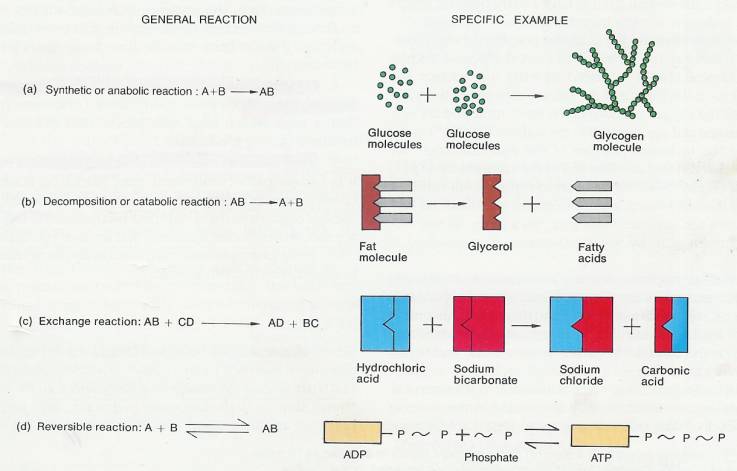
2.3.3 Inorganic Compounds
2.3.3.1 Water
2.3.3.1.1 One of
the most important, as well as the most abundant, inorganic substances in the
human organism is water. In fact, with a few exceptions, such as tooth enamel
and bone tissue, water is by far the most abundant material in tissues. About
60 percent of red blood cells, 75 percent of muscle tissue, and 92 percent of
blood plasma is water. The following functions of water explain why it is such
a vital compound in living systems:
2.3.3.1.1.1 Water is an
excellent solvent and suspending median. A solvent is a liquid or gas in which:
some oilier material (solid, liquid, or gas), called a solute, has been
dissolved. The combination of solvent plus solute is called a solution, and one
common example of a solution is salt water. A solute, such as salt when placed
in water, "'typically does not settle out of its solution. The solute can
be retrieved through a chemical reaction or, in some cases, by boiling off the
solvent. In a suspension, by contrast, the suspended material mixes with the
liquid or suspending medium, but it will eventually settle out of the mixture.
An example of a suspension is cornstarch and water. If the two materials are
shaken together, a milky mixture forms. After the mixture sits for a while,
however, the water clears at the top and the cornstarch settles to the bottom.
Since water serves as a solvent for so many solutes, it is referred to as the
universal solvent. .
2.3.3.1.1.2 The versatility of
water as a solvent is related to its polar covalent bonds. Recall that a
molecule of water contains polar covalent bonds, that is, bonds in which there
is an unequal sharing of electrons between atoms. One atom attracts the shared
electrons more strongly than others. In a molecule of water, there are both
positive and negative areas (Figure 2-6a). When the two hydrogen atoms bond
covalently to an oxygen atom, the shared electrons spend more time around
oxygen than hydrogen. Since electrons have a negative charge, the unequal
sharing causes the oxygen atom to have a slight negative charge and each
hydrogen atom to have a slight positive charge.
2.3.3.1.1.3 In order to
understand the solvating property of water, consider the following: If a
crystal of an ionic compound, such as sodium chloride (NaCL), is placed in
water, the sodium and chloride ions at the surface of the salt are exposed to
the water molecules. The oxygen portions of the water molecule are negatively
charged and are attracted to the sodium ion (Na +) of the salt, while the
hydrogen portions of the water molecule are positively charged and are
attracted to the chloride ions (Cl-) of the salt (Figure 2-6b). As
the pattern repeats itself from the surface of the salt inward, water molecules
surround the (Na +) and Cl- ions and separate them from each other. In this
way, the salt is taken apart by water molecules-it is dissolved in water.
2.3.3.1.1.4 The solvating
property of water is essential to health and survival. For example, if the
surfaces of the air sacs in your lungs are not moist, oxygen cannot dissolve
and therefore cannot move into your blood to be distributed throughout your
body. Water, moreover, is "the solvent that carries nutrients into and
wastes out of your body cells.
2.3.3.1.1.5 As a suspending
medium, water is also vital to your survival. Many large organic molecules are
suspended in the water of your body cells. These molecules are consequently
able to come in contact with other chemicals, allowing various essential
chemical reactions to occur.
2.3.3.1.1.6 Water can
participate in chemical reactions
2.3.3.1.1.6.1 During digestion, for example, water can be
added to large nutrient molecules in order to break them down into smaller
molecules. This kind of breakdown is necessary if the body is to utilize the
energy in nutrients. Water molecules are also used in synthesis reactions. Such
reactions occur in the production of hormones and enzymes.
2.3.3.1.1.7 Water absorbs and
releases heat very slowly
2.3.3.1.1.7.1 In
comparison to other substances, water requires a large amount of heat to
increase its temperature and a great loss of heat to decrease its. temperature.
Thus, the presence of a large amount of water moderates the effects of
fluctuations in environmental temperature and thereby helps to maintain a
homeostatic body temperature.
2.3.3.1.1.8 Water requires a
large amount of heat to change from a liquid to a gas
2.3.3.1.1.8.1 When
water (perspiration) evaporates from the skin, it takes with it large
quantities of heat and provides an excellent cooling mechanism.
2.3.3.1.1.9 Water serves as a
lubricant in various regions of the body
2.3.3.1.1.9.1 It
is a major part of mucus and other lubricating fluids. Lubrication is
especially necessary in the chest and abdomen, where internal organs touch and
slide over each other. It is also needed at joints, where bones, ligaments, and
tendons rub against each other. In the gastrointestinal tract, water in mucus
moistens foods to ensure their smooth passage
2.3.3.2
Figure 2-6
2.3.3.2.1 Solvating
property of water. (a) Polar covalent bonds in a molecule of water. (b) The
negative oxygen portions of the water molecules are attracted to the positive
sodium ions (Na+), while the positive hydrogen portions of the water
molecules-are attracted to the negative chloride ions (C-).
2.3.3.2.2 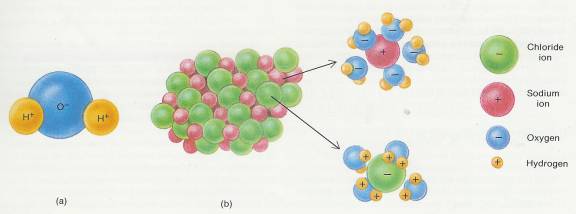
2.3.3.3 Inorganic Acids, Bases, and Salts
2.3.3.3.1 When
molecules of inorganic acids, bases, or salts are dissolved in water in the
body cells, they undergo ionization (ī-on-i-ZĀ-shun) or dissociation
(dis'-sō-sē-Āshun); that is, they dissociate (separate) into ions.
Such particles are also called electrolytes (ē-LEK-trō-līts)
because the solution will conduct an electric current (the chemistry and
importance of electrolytes are discussed in detail in Chapter 27). An acid may
be defined as a substance that dissociates into one or more hydrogen ions (H+)
and one or more negative ions (anions). Since an H + ion is a single proton
with a charge of + 1, an acid may also be defined as a proton donor. A base, by
contrast, dissociates into one or more hydroxyl ions (OH-) and one or more
positive ions (cations). A base may also be viewed as a proton acceptor.
Hydroxyl ions, as well as some other negative ions, have a strong attraction
for protons. A salt, when dissolved in water, dissociates into cations and
anions, neither of which is H+ or OH- (Figure 2-7). Acids and bases
react with one another to form salts. For example, the combination of
hydrochloric acid (HCI), an acid, and sodium hydroxide (NaOH), a base, produces
sodium chloride (NaCl), a salt, and water (H2O ).
2.3.3.3.2 Many
salts are found in the body. Some are in cells, whereas others are in the body
fluids, such as lymph, blood, and the extracellular fluid of tissues. The ions,
of salts are the source of many essential chemical elements. Exhibit 2-1 shows
how salts dissociate into ions that provide these elements. Chemical analyses
reveal that sodium and chloride ions are present in higher concentrations than
other ions in extracellular body fluids. Inside the cells, phosphate and
potassium ions are more abundant than other ions. Chemical elements such as
sodium, phosphorus, potassium, or iodine are present in the body only in
chemical combination with other elements or as ions. Their presence as free,
un-ionized atoms could be instantly fatal. Exhibit 2-2 lists representative
elements found in the body.
2.3.3.4
Exhibit 2-1
2.3.3.4.1 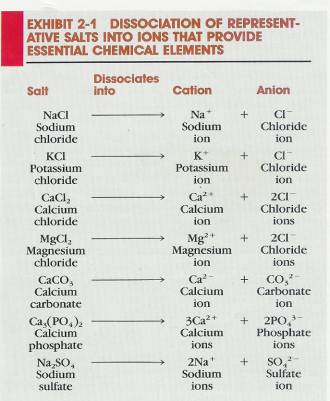
2.3.3.5
Figure 2-7
2.3.3.5.1 Ionization
of inorganic acids, bases, and salts. (a) When placed in water, hydrochloric
acid (HCI) dissociates into H + ions and CI- ions. Acids are proton donors. (b)
When the base sodium hydroxide (NaOH) is placed in water, it dissociates into
OH- ions and Na+ ions. Bases are proton acceptors. (c) When table salt (NaCI)
is placed in water, it dissociates into positive and negative ions (Na + and
CI-), neither of which is H + or OH - .
2.3.3.5.2 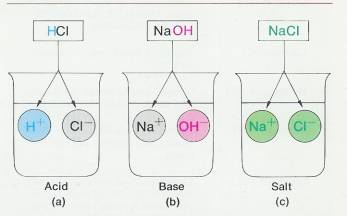
2.3.3.6 Acid-Base Balance: The Concept of pH
2.3.3.6.1 The
fluids of your body must maintain a fairly constant balance of acids and bases.
In solutions such as those found in body cells or in extracellular fluids,
acids dissociate into hydrogen ions (H +) and anions. Bases, on the other hand,
dissociate into hydroxyl ions (OH-) and cations. The more hydrogen ions that
exist in a solution, the more acid the solution; conversely, the more hydroxyl
ions, the more basic (alkaline) the solution. The term pH is
used to describe the degree of acidity
or alkalinity (basicity) of
a solution.
2.3.3.6.2
Biochemical reactions
2.3.3.6.2.1
Reactions that occur in living systems-are extremely
sensitive to even small changes in the acidity or alkalinity of the environment
in which they occur. In fact, H+ and OH- ions are involved in practically all
biochemical processes, and the functions of cells are modified greatly by any
departure from narrow limits of normal H+ and OH- concentrations. For this
reason, the acids and bases that are constantly formed in the body must be kept
in balance. .
2.3.3.6.3 A
solution's acidity or alkalinity is expressed on a pH scale
that runs from 0 to 14 (Figure 2-8). The pH scale is based on the
number of H+ ions in a solution expressed in chemical units called moles per liter. A pH of 7 means
that a solution contains one ten-millionth (0.0000001) of a mole* of H+ ions
per liter. The number 0.0000001 is written 10-7 in exponential form.
To convert this value to pH, the negative exponent ( - 7) is converted into the
positive number 7. A solution with a concentration of 0.0001 (10-4)
of H+ ions per liter has a pH of 4; a solution with a concentration
of 0.000000001 (10-9) has a pH of 9; and so on.
2.3.3.6.4 Exhibit
2-2
2.3.3.6.4.1 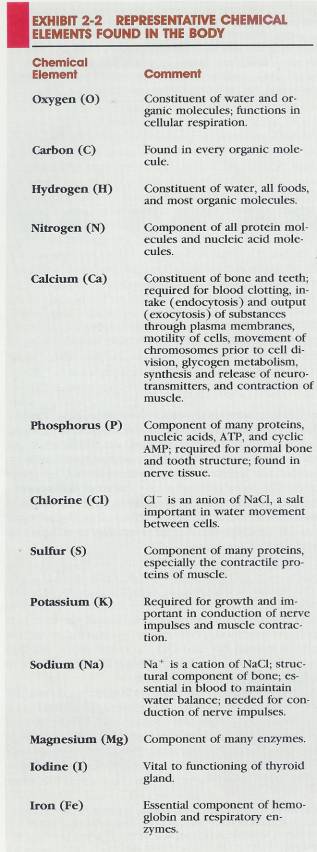
2.3.3.6.5 A
solution that is zero on the pH scale has many H+ ions and few
OH - ions. A solution that rates 14, by contrast, has
many OH- ions and few H+ ions. The midpoint in the scale is 7, where the
concentration of H+ and OH ions is equal. A substance with a pH of 7, such as
pure water, is neutral. A solution that has more H+ ions than OH- ions is an acid
solution and has a pH below 7. A solution that has more OH- ions than H+
ions is a basic (alkaline) solution and has a pH above 7. A change of one
whole number on, the pH scale represents a 10 fold change from the previous
concentration; that is, a pH of 2 indicates 10 times fewer H + ions than a pH
of 1. A pH of 3 indicates 10 times fewer H + ions than a pH
of 2 and 100 times fewer H+ ions than a pH of 1.
2.3.3.6.6 * A mole
of any substance is the weight, in grams, of the combined atomic
weights of the atoms that make up a molecule of the substance. Example: A mole
of H2O weighs 18 grams (2 for tl1e tWo hydrogen
atoms + 16 for the oxygen atom).
2.3.3.7 Maintaining pH: Buffer Systems
2.3.3.7.1 Although
the pH of body fluids may differ, the normal limits for the various fluids are
generally quite specific and narrow. Exhibit 2-3 shows the pH values for
certain body fluids compared with common substances. Even though strong acids
and bases are continually taken into the body, the pH levels of these body
fluids remain relatively constant. The mechanisms that maintain these homeostatic
pH values in the body are called buffer systems. .
2.3.3.7.2 The
essential function of a buffer system is to react with strong acids or bases in
the body and replace them with weak acids or bases so that the strong acids or
bases do not alter pH drastically. Strong acids (or bases) ionize easily and
contribute many H+ (or OH-) ions to a solution. They therefore change the pH
drastically. Weak acids (or bases) do not ionize so easily. They contribute
fewer H + (or OH -) ions and have little effect on the pH. The chemicals
that replace strong acids or bases with weak ones are called buffers
and are found in the body's fluids. Most buffers in the human body consist
of a weak acid and the salt of that acid. It is very important to note that the
salt of the acid functions as a weak base. At this point, we will examine the carbonic
acid--bicarbonate buffer system-the most important one found in
extracellular fluid.
2.3.3.7.3 Figure
2-8
2.3.3.7.3.1 FIGURE 2-8 pH
scale. At pH 7 (neutrality), the concentration of H+ and OH- ions is equal. A
pH value below 7 indicates an acid solution; that is, there are more H+ ions
than OH- ions. The lower the numerical value of the pH, the more acid the
solution is because the H+ ion concentration becomes progressively greater. A
pH value above 7 indicates an alkaline (basic) solution; that is, there are
more OH- ions than H+ ions. The higher the numerical value of the pH,
the more alkaline the solution is because the OH- ion concentration becomes
progressively greater. A change in one whole number on the pH scale represents
a 10-fold change from the previous concentration (100 = 1.0,10-1
= 0.1. 10-2 = 0.01. 10-3 = 0.001. and so on).
2.3.3.7.3.2 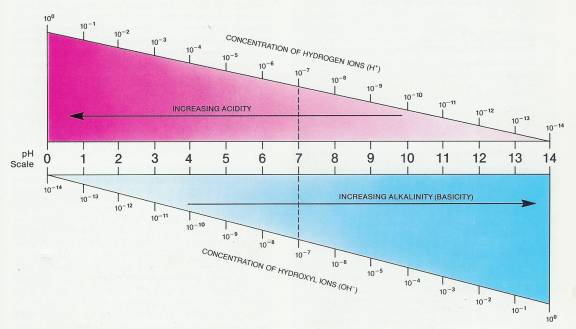
2.3.3.7.4 The
carbonic acid-bicarbonate buffer system consists of a pair of chemicals: carbonic
acid (H2CO3), which
functions as the weak acid, and sodium
bicarbonate (NaHCO3), the
salt of the weak acid, which functions as the weak base. (As you will
see in Chapter 27, it is actually the anion of the salt that behaves like the
weak base.) The carbonic acid is a proton donor, that is, a hydrogen ion (H +)
donor, and the bicarbonate ion (HCO3
-) of the sodium bicarbonate is a proton acceptor, that is, a hydrogen ion (H
+) acceptor. In solution, the members of this buffer pair dissociate as
follows:
2.3.3.7.5 Illustration
Weak Acid Component & Salt of the Acid Component
2.3.3.7.5.1 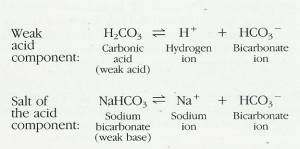
2.3.3.7.6 Each
member of the buffer pair has a specific role in helping the body maintain a
constant pH. If the body's pH is threatened by the presence of a strong acid,
the salt of the acid of the buffer pair behaves like a weak base and goes into
operation. If the body's pH is threatened by a strong base, the weak acid goes
into play.
2.3.3.7.7 Consider
the following situation. If a strong acid, such as HCI, is added to
extracellular fluid, the salt of the acid of the buffer system behaves like a
weak base and goes to work, and the following acid-buffering reaction occurs:
2.3.3.7.8 HCI
Hydrochloric Acid
2.3.3.7.8.1 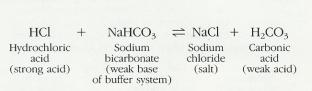
2.3.3.7.9 The chloride
ion (Cl-) of HCI and the sodium ion (Na +) of sodium bicarbonate combine to
form NaCl, a substance that has no effect on pH. The hydrogen ion of the HCI
could greatly lower pH by making the solution more acid, but this H + ion combines
with the bicarbonate ion (HCO3
-) of sodium bicarbonate to form carbonic acid, a weak acid that lowers pH only
slightly. In other words, because of the action of the salt of the acid of the
buffer system functioning as a weak base, the strong acid (HCI) has been replaced
by a weak acid (H2CO3) and a salt (NaCl),
and the pH remains relatively constant.
2.3.3.7.10
Now suppose a strong base, such as sodium hydroxide (NaOH), is
added to the extracellular fluid. In this instance, carbonic acid, the weak
acid of the buffer system, goes to work and the following base-buffering
reaction takes place
2.3.3.7.11
Exhibit 2-3
2.3.3.7.11.1
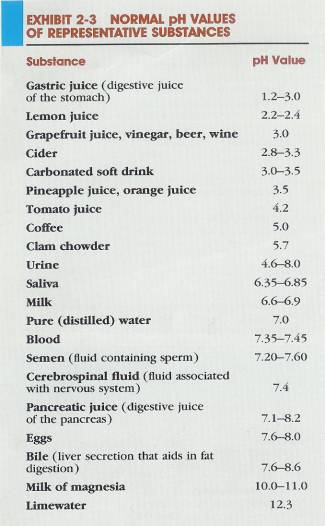
2.3.3.7.12
Illustration Base-Buffering Reaction
2.3.3.7.12.1
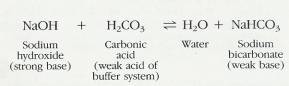
2.3.3.7.13
In this reaction, the OH- ion of sodium hydroxide could
greatly raise the pH of the solution by making it more alkaline. However, the
OH- ion combines with an H+ ion of carbonic acid and forms water, a substance
that has no effect on pH. In addition, the Na + ion
of sodium hydroxide combines with the bicarbonate ion (HCO3
-) to form sodium bicarbonate, a salt of the acid that acts like a weak base
and has little effect on pH. Thus, because of the action of the buffer system,
the strong base (NaOH) is replaced by water and a salt of the acid (NaHCO3),
that functions like a weak base, and the pH remains relatively constant.
2.3.3.7.14
Whenever a buffering reaction occurs, the concentration of
one member of the buffer pair is increased, whereas the concentration of the
other decreases. When a strong acid is buffered, for example, the concentration
of carbonic acid is increased, but the concentration of sodium bicarbonate is
decreased. This happens because carbonic acid is produced and sodium
bicarbonate is used up in the acid-buffering reaction. When a strong base is
buffered, the concentration of sodium bicarbonate is increased, but the
concentration of carbonic acid is decreased because sodium bicarbonate is
produced and carbonic acid is used up in the base-buffering reaction. When the
buffered substances-HCI and NaOH, in this case-are removed from the body via
the kidneys, the carbonic acid and sodium bicarbonate formed as products of the
reactions function again as components of the buffer pair. Do you now
understand why buffers are sometimes called "chemical sponges"?
2.3.3.7.15
Although the preceding discussion of pH has focused on
inorganic acids and bases, you should know that there are also organic acids
and bases and that they, too, are involved in counteracting potential pH
problems
2.3.4 Organic Compounds
2.3.4.1
In addition to carbon, the most
frequently found elements in organic compounds are hydrogen (which can form one
bond), oxygen (two bonds), and nitrogen (three bonds). Sulfur (two bonds) and
phosphorus (five bonds) appear less often. Other elements are found, but only
in a relatively few organic compounds. Carbon has several properties that
make it particularly useful to living organisms. For one thing, it can react
with one to several hundred other carbon atoms to form large molecules of many
different shapes. This means that the body can build many compounds out of
carbon, hydrogen, and oxygen. Each compound can be especially suited for a
particular structure or function. The relatively large size of most carbon
containing molecules and the fact that some do not dissolve easily in water
make them useful materials for building body structures. Carron compounds are
mostly or entirely held together by covalent bonds and tend to decompose
easily. This means that organic compounds are also a good source of energy.
Ionic compounds are not good energy sources because they form new ionic bonds
as soon as the old ones are broken.
2.3.4.2
Carbohydrates
2.3.4.2.1 A
large and diverse group of organic compounds found in the body are the carbohydrates,
also known as sugars and starches. The carbohydrates perform a number
of major functions in living systems. A few even form structural units. For
instance, one type of sugar (deoxyribose) is a building block of
deoxyribonucleic acid (DNA),'the molecule that carries hereditary information.
Some carbohydrates are converted to other substances, which are used to build
structures and provide an emergency source of energy. Other
carbohydrates function as food reserves. One example is glycogen, which is
stored in the liver and skeletal muscles. The principal function of
carbohydrates, however, is to provide the most readily available source of
energy to sustain life.
2.3.4.2.2 Carbon,
hydrogen, and oxygen are the elements found in carbohydrates. The ratio of
hydrogen to oxygen atoms is typically 2: 1, the same as in water. This ratio can
be seen in the formulas for carbohydrates such as ribose (CH5H10O5),
glucose (C6H12O6), and sucrose (C12H22011).
Although there are exceptions, the general formula for carbohydrates is (CH2O)n,
where n symbolizes three or more CH2O units. Carbohydrates can
be divided into three major groups on the basis of size: monosaccharides, disaccharides,
and polysaccharides.
2.3.4.2.2.1 Monosaccharides
2.3.4.2.2.1.1
Monosaccharides
(mon-ō-SAKa-rīds), or simple
sugars, are compounds containing from three to seven carbon atoms. Simple
sugars with three carbons in the molecule are called trioses. The number of
carbon atoms in the molecule is indicated by the prefix tri. There are
also tetroses (four-carbon sugars), pentoses (five-carbon sugars), hexoses
(six-carbon sugars), and heptoses (seven-carbon sugars). Pentoses and hexoses
are exceedingly important to the human organism. The pentose called
deoxyribose is a component of genes.
The hexose called glucose is the main energy-supplying molecule of the
body.
2.3.4.2.2.2 Disaccharides
2.3.4.2.2.2.1
A second group
of carbohydrates, the disaccharides (dī-SAK-a-rīds),
are also sugars and consist of two monosaccharides joined chemically. In the
process of disaccharide formation, two monosaccharides combine to form a
disaccharide molecule and a molecule of water is lost. This reaction is known
as dehydration synthesis (dehydration = loss
of water). The following reaction shows disaccharide formation. Molecules of
the monosaccharides glucose and fructose combine to form a molecule of the
disaccharide sucrose (table sugar):
2.3.4.2.2.2.2
Illustration
Disaccharide Formation
2.3.4.2.2.2.2.1

2.3.4.2.2.2.3 You
may be puzzled to see that glucose and fructose have the same chemical
formulas. Actually, they are different monosaccharides, since the relative
positions of the oxygens and carbons vary in the two different molecules (see
Figure 2-9). The formula for sucrose is C12H22011
and not C12H24012, since a molecule of H2O
is lost in the process of disaccharide formation. In every dehydration
synthesis, a molecule of water is lost. Along with this water loss, there is
the synthesis of two small molecules, such as glucose and fructose, into one
large, more complex molecule, such as sucrose (Figure 2-9). Similarly, the.
dehydration synthesis of the two monosaccharides glucose and galactose forms
the disaccharide lactose (milk sugar).
2.3.4.2.2.2.4 Disaccharides can also be broken down into
smaller, simpler molecules by adding water. This reverse chemical reaction is
called digestion (hydrolysis), which means to split by using
water. A molecule of sucrose, for example, may be digested into its components
of glucose and fructose by the addition of water. The mechanism of this
reaction also is represented in Figure 2-9,
2.3.4.2.2.3 Polysaccharides
2.3.4.2.2.3.1 The third major group of carbohydrates, the polysaccharides
(pol' -ē-SAK-a-rīds), consists of several monosaccharides
joined together through dehydration synthesis. Polysaccharides have the
formula (C6HIOO5)n. Like
disaccharides, polysaccharides can be broken down into their constituent sugars
through hydrolysis reactions. Unlike monosaccharides or disaccharides,
however, they usually lack the characteristic sweetness of sugars like fructose
or sucrose and are usually not soluble in water. One of the chief
polysaccharides is glycogen.
2.3.4.2.3 Clinical
Applications: Artificial Sweeteners
2.3.4.2.3.1 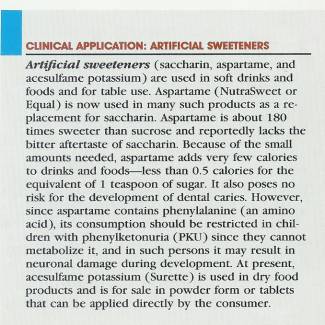
2.3.4.2.4 Figure 2-9
2.3.4.2.4.1 FIGURE 2-9 Dehydration
synthesis and hydrolysis of a molecule of sucrose. In the dehydration synthesis
reaction (read from left to right), the two smaller molecules, glucose and
fructose, are joined to form a larger molecule of sucrose. Note the loss of a
water molecule. In hydrolysis (read from right to left), the larger sucrose
molecule is broken down into the two smaller molecules, glucose and fructose.
Here, a molecule of water is added to sucrose for the reaction to occur.
2.3.4.2.4.2 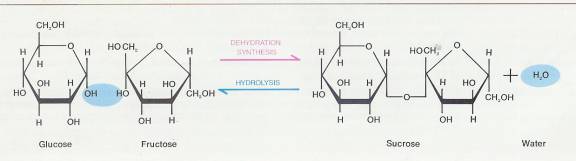
2.3.4.2.4.3
2.3.4.3
Lipids
2.3.4.3.1 A
second group of organic compounds that is vital to
the human organism is the lipids.
Like carbohydrates, lipids are composed of carbon, hydrogen, and
oxygen, but they do not have a 2:1 ratio of hydrogen to oxygen. In fact, the
amount of oxygen in lipids is usually less than that in carbohydrates. Most
lipids are insoluble in water, but they readily dissolve in solvents such as
alcohol, chloroform, and ether. Among the groups of lipids are fats, phospholipids
(lipids that contain phosphorous), steroids, carotenes, vitamins E and K, and
prostaglandins (PGs). A listing of the various types of lipids is shown in
Exhibit 2-4, along with their relationships to the human organism. Since lipids
are a large and diverse group of compounds, we will discuss only two types in
detail at this point: fats and prostaglandins.
2.3.4.3.2 A
molecule of fat (triglyceride) consists of two basic components: glycerol and
fatty acids (Figure 2-10). A
single molecule of fat is formed when a molecule of glycerol combines with
three molecules of fatty acids. This reaction, like the one described for
disaccharide formation, is a dehydration synthesis reaction. During
hydrolysis, a single molecule of fat is broken down into fatty acids and
glycerol.
2.3.4.3.3 Exhibit
2-4
2.3.4.3.3.1 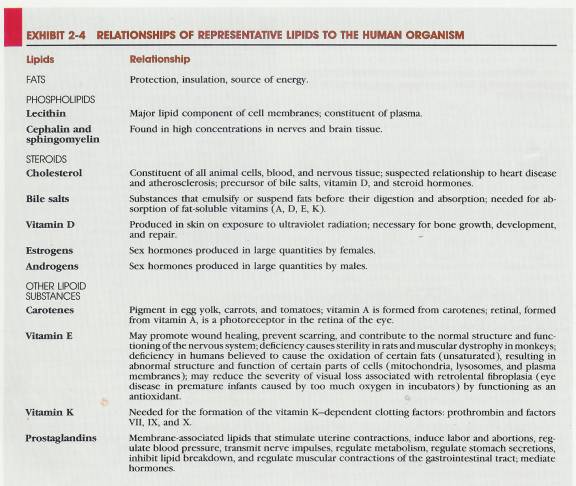
2.3.4.3.4 In
subsequent chapters, we will be talking about saturated, monosaturated, and
polyunsaturated fats. These terms have the following meanings. A saturated fat
contains no double bonds between any of its carbon atoms. It contains only
single covalent bonds between its carbon atoms, and all the carbon atoms are
bonded to the maximum number of hydrogen atoms; thus, such a fat is saturated
with hydrogen atoms (Figure 2-10c). Saturated fats (and some cholesterol) occur
mostly in animal foods such as beef, pork, butter, whole milk, eggs, and
cheese. They also occur in some plant products such as cocoa butter, palm oil,
and coconut oil. Since the liver uses some breakdown products of saturated fats
to produce cholesterol, consumption of these fats is discouraged for
individuals with high cholesterol levels. A monosaturated fat contains one
double covalent bond between its carbon atoms; it is not completely saturated
with hydrogen atoms (Figure 2-10c). Examples are olive oil and peanut oil,
which are believed to help reduce cholesterol levels. A polyunsaturated fat
contains more than one double covalent bond between its carbon atoms (Figure
2-10c). Corn oil, safflower oil, sunflower oil, cottonseed oil, sesame oil, and
soybean oil are examples of polyunsaturated fats, which researcher believe also
help to reduce cholesterol in the blood.
2.3.4.3.5 Fats
represent the body’s most highly concentrated source of energy.
2.3.4.3.6 They
provide more than twice as much energy per-weight as either carbohydrates or
proteins. In addition, fats do not attract water and thus do not result in
excessive water retention in the body. In general, however, fats are about 10
to 12 percent less efficient as body fuels than are carbohydrates. A great
amount of the fat calorie is wasted and thus not available for the body to use.
2.3.4.3.7 FIGURE 2-10
2.3.4.3.7.1 Structure and
reactions of glycerol and fatty acids. (a) Structure of glycerol. (b) Structure
of a fatty acid. The one shown here is palmitic acid. Note that when glycerol
and the fatty acid are joined in dehydration synthesis, a molecule of water is
lost. (c) Fats consist of one molecule of glycerol joined to three molecules of
fatty acids, which vary in length and the number and location of double bonds
between carbon atoms (C=C). Shown here is a molecule of a fat that
contains three different fatty acids: palmitic acid, a saturated fatty acid;
lineolic acid, a polyunsaturated fatty acid; and oleic acid, a monosaturated
fatty acid. Note that when a molecule of glycerol combines with three fatty
acids in dehydration synthesis to form a molecule of fat, three molecules of
water are lost.
2.3.4.3.7.2
2.3.4.3.8 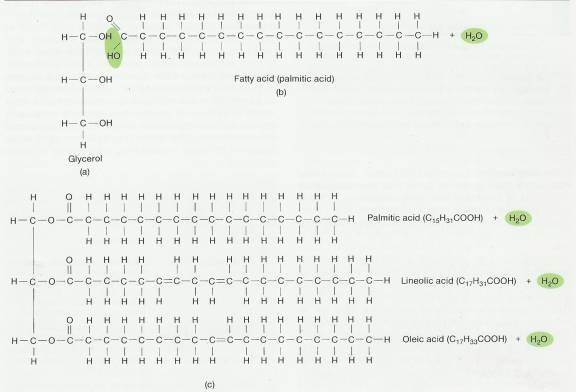
2.3.4.3.9 Clinical
Application
2.3.4.3.9.1 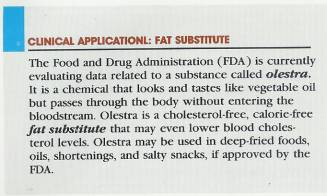
2.3.4.3.10
Prostaglandins (pros'-ta-GLAN-dins), also called PGs, are a large group of
membrane-associated lipids composed of 20-carbon fatty acids containing 5
carbon atoms joined to form a ring (cyclopentane ring). Prostaglandins are produced
in all nucleated cells in the body and are able to influence the functioning of
any type of cell.
2.3.4.3.11
Prostaglandins are produced in cell membranes and are rapidly
decomposed by catabolic enzymes. Although synthesized in minute quantities,
they are potent substances and exhibit a wide variety of effects on the body.
Basically, prostaglandins mimic hormones. They are involved in modulating many
hormonal responses (Chapter 18), inducing menstruation, and inducing
second-trimester abortions. They are also involved in contributing to the
inflammatory response (Chapter 22), preventing peptic ulcers, opening
bronchial and nasal passages, platelet aggregation and inhibition of
aggregation, and regulating body temperature.
2.3.4.4
Proteins
2.3.4.4.1 A
third principal group of organic compounds is proteins: These compounds are much more complex in structure
than the carbohydrates or lipids. They are also responsible for much of the
structure of body cells and are related to many physiological activities. For
example, proteins in the form of enzymes speed up most essential biochemical
reactions. This is described in detail in Chapter 25. Other proteins assume a
necessary role in muscular contraction. Antibodies are proteins that provide
the human organism with defenses against invading microbes. And some hormones
that regulate body functions are also proteins. A classification of proteins on
the basis of function is shown in Exhibit 2-5.
2.3.4.4.2 Chemically,
proteins always contain carbon, hydrogen, oxygen, and nitrogen. Many proteins
also contain sulfur and phosphorus. Just as monosaccharides are the building
units of sugars, and fatty acids and glycerol are the building units of fats, amino acids are the building blocks of
proteins. In protein formation, amino acids combine to form more complex
molecules, while water molecules are lost. The process is a dehydration
synthesis reaction, and the bonds formed between amino acids are called peptide bonds (Figure 2-11).
2.3.4.4.3
When two amino acids combine, a dipeptide results. Adding another
amino acid to a dipeptide produces a tripeptide.
Further additions of amino acids result in the formation of chainlike polypeptides, which are large
protein molecules that consist of 50 or more amino acids. At least 20 different
amino acids are found in proteins. Although all amino acids have one component
in common, each is distinguished on the basis of additional atoms or groups of
atoms arranged in a specific way. A great variety of proteins are possible
because each variation in the number or sequence of amino acids can produce a
different protein. The situation is similar to using an alphabet of 20 letters
to form words. Each letter could be compared to a different amino acid, and each
word would be a different protein.
2.3.4.4.4 Exhibit
2-5
2.3.4.4.4.1 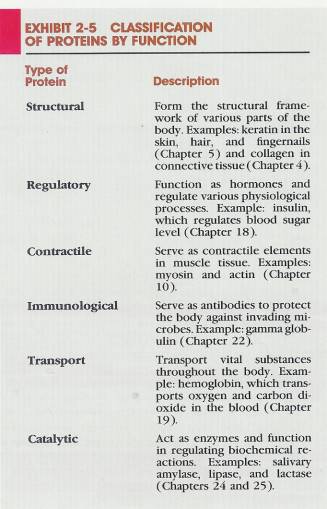
2.3.4.4.5 Figure
2-11
2.3.4.4.5.1 Protein formation.
When two or more amino acids are chemically united, the resulting bond between
them is called a peptide bond. In the example shown here, the amino acids
glycine and alanine are joined to form the dipeptide glycylalanine. The peptide
bond is formed at the point where water is lost.
2.3.4.4.5.2 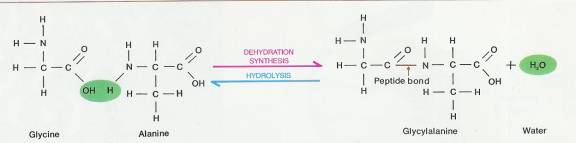
2.3.4.4.6 Proteins
vary tremendously in structure. Different proteins have different
architectures and different three-dimensional shapes. This variation in structure
and shape is directly related to their diverse functions. When a cell makes a
protein, the polypeptide chain folds spontaneously to assume a certain shape.
One reason for folding of the polypeptide is that some parts of a protein are
attracted to water, and other parts are repelled by it. In practically every
case, the function of a protein depends on its ability to recognize and bind to
some other molecule, a situation similar to the fit between a lock and key; As
an example, an enzyme binds specifically with its substrate. A hormonal protein
binds to a receptor on a cell whose function it will alter. An antibody binds
to a foreign substance (antigen) that has invaded the body. The unique shape of
a protein permits it to interact with other specific molecules in order to
carry out specific functions.
2.3.4.4.7 Proteins
exhibit four levels of structural organization. The primary structure is the
unique order (sequence) of amino acids making up the protein. An alteration in primary
structure can have serious consequences. For example, a single substitution of
an amino acid in a blood protein can result in a deformed hemoglobin molecule
that produces sickle-cell anemia. The secondary structure of a protein is the
localized, repetitious twisting or folding of its polypeptide chain. Common
secondary structures are clockwise spirals (helixes) and pleated sheets. The
tertiary structure refers to the overall three-dimensional structure of a
polypeptide chain. The folding is not repetitive or predictable as in secondary
structures. The tertiary structure is very irregular, and it determines the
particular function of a protein. The quaternary
structure of a protein refers to two or more individual polypeptide
chains bonded to each other that function as a single unit.
2.3.4.4.8
It was once thought that the atoms in proteins are in
fixed positions and that proteins are therefore rigid molecules. However,
based on computer simulations, it has been learned that the atoms in proteins
are in constant motion, usually twisting movements. Such motion is due to the
chemical bonds between atoms that act like springs. The internal motion of
proteins results in their constantly changing structure and explains the many
and varied functions of proteins. Scientists are now studying how proteins
fold into three-dimensional structures that determine their biological
functions.
2.3.4.4.9 If a
protein encounters a hostile environment in terms of temperature, pH, or salt
concentrations, it may unravel and lose its characteristic shape. This process
is called denaturation. As a result
of denaturation, the protein is no longer functional.
2.3.4.4.10
Nucleic Acids: Deoxyribonucleic Acid (DNA) and Ribonucleic
Acid (RNA)
2.3.4.4.10.1
Nucleic (noo-KLĒ-ic) acids, compounds first
discovered in the nuclei of cells, are exceedingly large organic molecules
containing carbon, hydrogen, oxygen, nitrogen, and phosphorus. They are divided
into two principal kinds: deoxyribonucleic (dē-ok'
-sē-rī' -bō-noo-KLĒ-ik) acid (DNA) ,:and ribonucleic
acid (RNA). I
2.3.4.4.10.2
Whereas the basic structural units of proteins are amino
acids, the basic units of nucleic acids are nucleotides. A molecule of DNA is a
chain composed of repeating nucleotide units. Each nucleotide of DNA consists
of three basic parts (Figure 2-12a):
2.3.4.4.10.2.1
It contains
one of four possible nitrogenous bases, which are ring-shaped structures
containing atoms of C, H, O, and N. The nitrogenous bases found in DNA are
adenine, Thymine, cytosine, and guanine. Adenine and guanine are double-ring
structures, collectively referred to as purines. Thymine and cytosine
are smaller, single-ring structures called pyrimidines.
2.3.4.4.10.2.2
It contains
a pentose sugar called deoxyribose.
2.3.4.4.10.2.3
It also
contains phosphate groups.
2.3.4.4.10.3
The nucleotides are named according to the nitrogenous base
that is present. Thus, a nucleotide containing thymine is called a thymine
nucleotide. One containing adenine is called an adenine nucleotide, and
so on.
2.3.4.4.10.4
The chemical components of the DNA molecule were known before
1900, but it was not until 1953 that a model of the organization of the
chemicals was constructed. This model was proposed by J. D. Watson and F. H. C.
Crick on the basis of data from many investigations. Figure 2-12b shows the
following structural characteristics of the DNA molecule.
2.3.4.4.10.4.1
The molecule consists of two strands with crossbars. The
strands twist about each other in the form of a double helix, so
that the shape resembles a twisted ladder. For many years, it was assumed that
all DNA was in the form of a double helix that twisted smoothly to the right
(right-handed DNA). There at least four varieties of right-handed DNA
designated as A-DNA, B-DNA, C-DNA, and D-DNA. Watson and Crick's model, the one
most commonly found in cells, is B-DNA. It was discovered, however, that DNA
can also twist jaggedly to the left (Z-DNA or left-handed DNA). This alternate
form of DNA is located near the ends of genes and may help to explain how genes
turn on and off and how some cells may become malignant.
2.3.4.4.10.4.2
The uprights of the DNA
ladder consist of alternating phosphate groups and the deoxyribose portions of
the nucleotides.
2.3.4.4.10.4.3
. The rungs ofthe
ladder contain paired nitrogenous bases. As shown, adenine always pairs with
thymine, and cytosine always pairs with, guanine.
2.3.4.4.10.5
Figure 2-12
2.3.4.4.10.5.1
DNA molecule. (a) Adenine nucleotide. (b) Portion of an
assembled DNA molecule. (c) Strand of DNA viewed through a scanning tunneling
microscope. This is the first direct image of chemically unaltered, uncoated,
pure DNA. The looped DNA strand stretches across an area 400 A wide. The
circular structure is possibly an unresolved DNA fragment. (Courtesy of
Lawrence Livermore National Laboratory.)
2.3.4.4.10.5.2
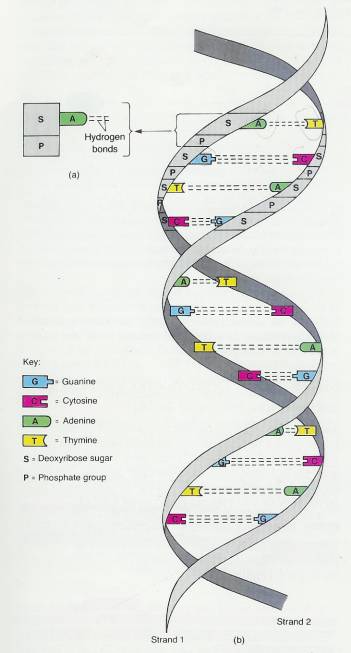
2.3.4.4.10.5.3
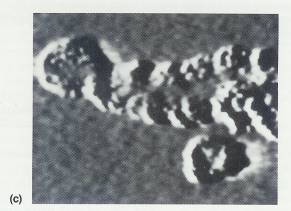
2.3.4.4.10.6
Cells contain hereditary material called genes, each of
which is a segment of a DNA molecule. Our genes determine which traits we
inherit, and they control all the activities that take place in our cells
throughout a lifetime. When a cell divides, its hereditary information is
passed on to the next generation of cells. The passing of information is
possible because of DNA's unique structure.
2.3.4.4.10.7
Clinical Application
2.3.4.4.10.7.1
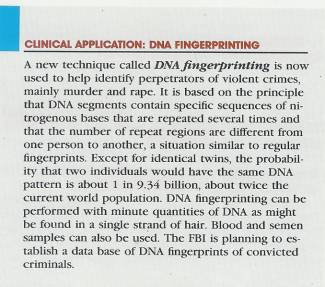
2.3.4.4.10.8
RNA, the second principal kind of nucleic acid, differs from
DNA in several respects. RNA is single-stranded; DNA is double-stranded. The
sugar in the RNA nucleotide is the pentose ribose. And RNA does not contain the
nitrogen base thymine. Instead of thymine, RNA has the nitrogen base
uracil. At least three different kinds of RNA have been identified in cells.
Each type has a specific role to perform with DNA in protein synthesis
reactions (Chapter 3).
2.3.4.5
Adenosine Triphosphate (ATP)
2.3.4.5.1 Figure
2-13
2.3.4.5.1.1 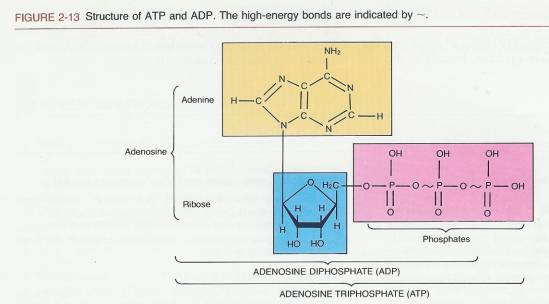
2.3.4.5.2 A
molecule that is indispensable to the life of the cell is adenosine (a-DEN-o-sen) triphosphate (ATP). This substance
is found universally in living systems and performs the essential function of
storing energy for various cellular activities. Structurally, ATP consists of
three phosphate groups (PO43-) and an adenosine unit
composed of adenine and the five-carbon sugar ribose (Figure 2-13). ATP is
regarded as a high-energy molecule because of the total amount of usable energy
it releases when it is broken down by the addition of a water molecule (hydrolysis
).
2.3.4.5.3 When
the terminal phosphate group (here symbolized by P) is hydrolyzed, the reaction
liberates a great deal of energy. This energy is used by the cell to perform
its basic activities. Removal of the terminal phosphate group leaves a molecule
called adenosine diphosphate (ADP). This reaction may be represented as
follows:
2.3.4.5.4 Illustration
ADP
2.3.4.5.4.1 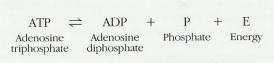
2.3.4.5.5 The
energy supplied by the catabolism of ATP into ADP is constantly being used by the
cell. Since the supply of ATP at any given time is limited, a mechanism exists
to replenish it-a phosphate group is added to ADP to manufacture more ATP. The
reaction may be represented as follows:
2.3.4.5.6 Illustration
ATP
2.3.4.5.6.1 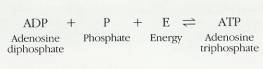
2.3.4.5.7 Logically,
energy is required to manufacture ATP. The energy required to attach a
phosphate group to ADP is supplied by various decomposition reactions taking
place in the cell, particularly by the decomposition of glucose. Glucose is
completely metabolized in cells into carbon dioxide and water, and the energy
released in this process is used to attach a phosphate group to ADP to
resynthesize ATP. ATP can be stored in every cell, where it provides potential
energy that is not released until needed.
2.3.4.6
Cyclic AMP
2.3.4.6.1 A
chemical substance closely related to ATP is cyclic AMP, also known as adenosine-3',5'-monophosphate. Essentially it is a molecule of
adenosine monophosphate with the phosphate attached to the ribose sugar at two
places (Figure 2-14). START
2.3.4.6.2 The attachment
forms a ring-shaped structure and thus the name cyclic AMP.
2.3.4.6.3 Cyclic
AMP is formed from ATP by the action of a special enzyme, called adenylate cyclase, located in the
cell membrane. Although cyclic AMP was discovered in 1958, only recently has its
function in cells become clear. One function is related to the action of
hormones, a topic we explore in detail in Chapter 18.
2.3.4.6.4 Figure
2-14
2.3.4.6.4.1 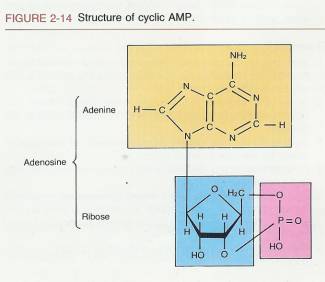
2.4 Summary
2.4.1 Introduction to Basic Chemistry (Link)
2.4.1.1
Chemical Elements (Link)
2.4.1.1.1 Matter
is anything that occupies space and has mass. It is made up of building units
called chemical elements.
2.4.1.1.2 Oxygen,
carbon, hydrogen, and nitrogen make up 96 percent of body weight. These
elements together with calcium and phosphorus make up 99 percent of total body
weight.
2.4.1.2
Structure
of Atoms (Link)
2.4.1.2.1 Units
of matter of all chemical elements are called atoms.
2.4.1.2.2 Atoms
consist of a nucleus, which contains protons and neutrons (nucleons), and
electrons that move about the nucleus in energy levels.
2.4.1.2.3 The
total number of protons of an atom is its atomic number. This number is equal
to the number of electrons in the atom.
2.4.1.3
Atoms
and Molecules (Link)
2.4.1.3.1 The
electrons are the part of an atom that actively participate in chemical
reactions.
2.4.1.3.2 A
molecule is the smallest unit of two or more combined atoms. A molecule
containing two or more different kinds of atoms is a compound.
2.4.1.3.3 In an
ionic bond, outer-energy-level electrons are transferred from one atom to
another. The transfer forms ions, whose unlike charges attract each other and
form ionic bonds.
2.4.1.3.4 In a
covalent bond, there is a sharing of pairs of outer-energy level electrons.
2.4.1.3.5 Hydrogen
bonding provides temporary bonding between certain atoms within large complex
molecules such as proteins and nucleic acids.
2.4.1.4
Chemical
Reactions (Link)
2.4.1.4.1 Synthesis
reactions involve the combination of reactants to produce a new molecule. The
reactions are anabolic: bonds are formed.
2.4.1.4.2 In decomposition
reactions, a substance breaks down into other substances. The reactions are
catabolic: bonds are broken.
2.4.1.4.3 Exchange
reactions involve the replacement of one atom or atoms by another atom or
atoms.
2.4.1.4.4 In
reversible reactions, end products can revert to the original combining
molecules.
2.4.1.4.5 When
chemical bonds are formed (endergonic reaction), energy is needed. When bonds
are broken (exergonic reaction), energy is released. This is known as chemical
bond energy.
2.4.1.4.6 Other
forms of energy include mechanical, radiant, and electrical.
2.4.2 Chemical Compounds and Life Processes (Link)
2.4.2.1
Inorganic substances usually lack
carbon, contain ionic bonds, resist decomposition, and dissolve readily in
water.
2.4.2.2
Organic substances always contain carbon
and hydrogen. Most organic substances contain covalent bonds and many are
insoluble in water.
2.4.2.3
Inorganic Compounds (p. 37)
2.4.2.3.1 Water
is the most abundant substance in the body. It is an excellent solvent and
suspending medium, participates in chemical reactions, absorbs and releases
heat slowly, and lubricates.
2.4.2.3.2 Inorganic
acids, bases, and salts dissociate into ions in water. An acid ionizes into H+
ions; a base ionizes into OH- ions. A salt ionizes into neither H+ nor OH-
ions. Cations are positively charged ions; anions are negatively charged ions.
2.4.2.3.3 The pH
of different parts of the body must remain fairly constant for the body to
remain healthy. On the pH scale, 7 represents neutrality. Values below 7 indicate
acid solutions, and values above 7 indicate alkaline solutions.
2.4.2.3.4 The pH
values of different parts of the body are maintained by buffer systems, which
usually consist of a weak acid and a weak base. Buffer systems
eliminate excess H+ ions and excess OH- ions in order to maintain pH
homeostasis.
2.4.2.4
Organic
Compounds (Link)
2.4.2.4.1 Carbohydrates
are sugars or starches that provide most of the energy needed
for life: They may be monosaccharides, disaccharides, or polysaccharides.
Carbohydrates, and other organic molecules, are joined together to form larger
molecules with the loss of water by a process called dehydration synthesis. In
the reverse process, called digestion (hydrolysis), large molecules are broken
down into smaller ones upon the addition of water.
2.4.2.4.2 Lipids
are a diverse group of compounds that includes fats, phospholipids, steroids,
carotenes, vitamins E and K, and prostaglandins (PGs). Fats protect, insulate,
provide energy, and are stored. Prostaglandins mimic the effects of hormones
and are involved in the inflammatory response and the modulation of hormonal
responses.
2.4.2.4.3 Proteins
are constructed from amino acids. They give structure to the body,
regulate processes, provide protection,; help muscles to contract, transport substances,
and serve as enzymes. Structural levels of organization among proteins include:
primary, secondary, tertiary, and quaternary.
2.4.2.4.4 Deoxyribonucleic
acid (DNA) and ribonucleic acid (RNA) are nucleic acids consisting of nitrogenous
bases, sugar, and phosphate groups. DNA is a double helix and is
the primary chemical in genes. RNA differs in structure and chemical
composition from DNA and is mainly concerned with protein synthesis reactions.
2.4.2.4.5 The
principal energy-storing molecule in the body is adenosine triphosphate (ATP).
When its energy is liberated, it is decomposed to adenosine diphosphate
(ADP) and P. ATP is manufactured from ADP and P using the energy supplied by
various decomposition reactions, particularly of glucose.
2.4.2.4.6 . Cyclic AMP is closely related to ATP and
assumes a function in certain hormonal reactions
4.1 Introduction
4.1.1 Cells are highly organized units, but, in multicellular organisms,
they do not function in isolation. They work together in a group of similar
cells called a tissue.
4.2 Types of Tissues
4.2.1 A tissue is a group of similar cells and their intercellular
substance that have a similar origin in an embryo and function together to
perform a specialized activity.
4.2.2 The science that deals with the study of tissues is called histology (hiss’-TOL-ō-jē;
histio=tissue; logos=study of). The various tissues of the body are classified
into four principal types according to their function and structure:
4.2.2.1
Epithelial
(ep'-i-T.HĒ-lē-al) tissue, which covers body surfaces, lines body
cavities and ducts, and forms glands.
4.2.2.2
Connective
tissue, which protects and supports the body and its organs, binds organs
together, and stores energy reserves.
4.2.2.3
Muscular
tissue, which is responsible for movement through the active generation of
force.
4.2.2.4
Nervous
tissue, which initiates, transmits, and interprets nerve impulses that
coordinate body activities.
4.2.3 Epithelial tissue and connective tissue, except for bone and blood,
will be discussed in detail in this chapter. The general features of bone
tissue and blood will be introduced here, but their detailed discussion occurs
later in the book. Similarly, the detailed discussion of muscle tissue and
nervous tissue will be postponed.
4.3 Epithelial Tissue
4.3.1 Epithelial tissues perform many activities in the body, ranging from
protection of underlying tissues against microbial invasion, drying out, and
harmful environmental factors to secretion. Epithelial tissue, or more simply, epithelium, may be divided into two
subtypes: (1) covering and lining
epithelium and (2) glandular epithelium. Covering and
lining epithelium forms the outer covering of external body surfaces and the
outer covering of some internal organs. It lines body cavities and the
interiors of the respiratory and gastrointestinal tracts, blood vessels, and
ducts. It makes up, along with nervous tissue, the parts of the sense organs
for smell, hearing, vision, and touch, which respond to stimuli. And it is the
tissue from which gametes (sperm and eggs) develop. Glandular epithelium
constitutes the secreting portion of glands.
4.3.2 Both types of epithelium consist largely or entirely of closely
packed cells with little or no intercellular material between adjacent cells.
(Such intercellular material is also called the matrix.) The points of attachment
between adjacent plasma membranes of epithelial cells are called cell junctions. They not only
provide cell-to-cell attachments but also inhibit the movement of materials
into certain cells and provide channels for communication between other cells.
Epithelial cells are arranged in continuous sheets that may be either single
or multilayered. Nerves may extend through these sheets, but blood vessels do
not. They are avascular (a = without; vascular = blood
vessels). The vessels that supply nutrients and remove wastes are located in
underlying connective tissue.
4.3.3 Both types of epithelium overlie and adhere firmly to the connective
tissue, which holds the epithelium in position and prevents it from being
torn. The attachment between the epithelium and the connective tissue is a thin
extracellular layer called the basement membrane. With few exceptions, epithelial cells secrete along their basal
surfaces a material consisting of a special type of collagen and glycoproteins.
This structure averages 50 to 80 nm in thickness and is referred to as the basal
lamina. Frequently, the basal lamina is reinforced by an underlying reticular lamina consisting of
reticular fibers and glycoproteins. This lamina is produced by cells in the
underlying connective tissue. The combination of the basal lamina and reticular
lamina constitutes the basement membrane.
4.3.4 Within a tissue, most normal cells remain in place, anchored to
basement membranes and connective tissues. In an adult, a few cells, such as
phagocytes, routinely move through the intercellular material (matrix) during
an infection. And, in an embryo, certain cells migrate extensively as part of
the growth and development process. Various activities of cells depend on a
variety of adhesion proteins found in the intercellular material and blood. These proteins
interact with receptors on plasma membranes called integrins. Many adhesion proteins
contain tripeptides (arginine, glycine, and aspartic acid) as the recognition
site for integrins. Among the adhesion proteins are fibronectin, vitronectin,
osteopontin, collagens, thrombospondin, and fibrinogen. Together, adhesion
proteins and their integrins function in anchoring cells in position, providing
traction for the movement of cells, differentiation, positioning of cells, and
possibly growth.
4.3.5 Some tissues of the body are so
highly differentiated (specialized) that they have lost their capacity for
mitosis. Examples are muscle tissue and nervous tissue. Other tissues, such as
epithelium, which are subjected to a certain amount of wear and tear and
injury, have a continuous capacity for renewal because they contain stem cells
that are capable of renewing the tissue. Examining cells that are sloughed off
provides the basis for the Pap smear, a test for
precancer and cancer diagnosis of the uterus, cervix, and vagina (Chapter 28).
4.3.6 Covering and Lining Epithelium
4.3.6.1 Arrangement of Layers
4.3.6.1.1 Covering
and lining epithelium is arranged in several different ways related to location
and function. If the epithelium is specialized for absorption or filtration
and is in an area that has minimal wear and tear, the cells of the tissue are
arranged in a single layer. Such an arrangement is called simple epithelium. If the epithelium
is found, in an area with a high degree of wear and tear, then the / cells
are stacked in several layers. This tissue is referred to as stratified epithelium. A third, less
common arrangement of epithelium is called pseudostratified columnar. Like simple epithelium,
pseudostratified epithelium has only one layer of cells. However, some of the
cells do not reach the surface-an arrangement that gives the tissue a
multilayered, or stratified, appearance. The cells in pseudostratified
epithelium that do reach the surface either secrete mucus or contain cilia that
move mucus and foreign particles for eventual elimination from the body.
4.3.6.2 Cell Shapes
4.3.6.2.1 In
addition to classifying covering and lining epithelium according to the number
of its layers, we may also categorize it by cell shape. The cells may be flat,
cube like, columnar or a combination of shapes. Squamous_(SKWĀ-mus)
cells are flattened and scale like. They are attached to each other and form a
mosaic pattern. Cuboidal
cells; are usually cube-shaped In cross section. They sometimes appear
as hexagons. Columnar
cells are tall and cylindrical, appearing as somewhat rectangular in
shape when set on end. Transitional
cells often have a combination of shapes and are found where there is a
great degree of distention or expansion in the body. Transitional cells in the
bottom layer of an epithelial tissue may range in shape from cuboidal to
columnar. In the intermediate layer, they may be cuboidal or polyhedral. In the
superficial layer, they may range from cuboidal to squamous, depending on how
much they are pulled out of shape during certain body functions.
4.3.6.3 Classification
4.3.6.3.1 Considering
layers and cell shapes in combination, we may classify covering and lining
epithelillum as follows:
4.3.6.3.1.1 Simple
4.3.6.3.1.1.1 Squamous
4.3.6.3.1.1.2 Cuboidal
4.3.6.3.1.1.3 Columnar
4.3.6.3.1.2 Stratified
4.3.6.3.1.2.1 Squamous
4.3.6.3.1.2.2 Cuboidal
4.3.6.3.1.2.3 Columnar
4.3.6.3.1.2.4 Transitional
4.3.6.3.1.3 Pseudostratified
columnar
4.3.6.3.1.3.1 Each
of the epithelial tissues described in the following sections is illustrated in
Exhibit 4-1
4.3.6.4 Simple Epithelium
4.3.6.4.1 Simple
Squamous Epithelium
4.3.6.4.1.1 This type of simple
epithelium consists of a single layer of flat, scale like cells. Its surface
resembles a tiled floor. The nucleus of each cell is centrally located and oval
or spherical. Since simple squamous epithelium has only one layer of cells, it
is highly adapted to diffusion, osmosis, and filtration. Thus, it lines the air
sacs of the lungs, where respiratory gases (oxygen and carbon dioxide) are
exchanged between air spaces and blood. It is present in the part of the kidney
that filters blood. It also lines the inner surfaces of the membranous
labyrinth and tympanic membrane of the ear. Simple squamous epithelium is found
in body parts that have little wear and tear.
4.3.6.4.1.2 Simple squamous
epithelium that lines the heart, blood vessels, and lymph vessels and forms the
walls of capillaries is known as endothelium. Simple squamous epithelium
that forms the epithelial layer of serous membranes is called mesothelium. Serous
membranes line the thoracic and abdominopelvic cavities and cover the viscera
within them.
4.3.6.4.2 Simple
Cuboidal Epithelium
4.3.6.4.2.1 Viewed from above,
the cells of simple cuboidal epithelium appear as closely fitted polygons. The
cuboidal nature of the cells is obvious only when the tissue is sectioned at
right angles. Like simple squamous epithelium, these cells possess a central
nucleus that is usually round. Simple cuboidal epithelium covers the surface of
the ovaries, lines the anterior surface of the capsule of the lens of the eye,
and forms the pigmented epithelium of the retina of the eye. In the kidneys, where
it forms the kidney tubules and contains microvilli, it functions in water
reabsorption. It also lines the smaller ducts of some glands and the secreting
units of glands, such as the thyroid.
4.3.6.4.2.2 Simple cuboidal
epithelium performs the functions of secretion and absorption. Secretion is
the production and release by cells of a fluid that may contain a variety of
substances such as mucus, perspiration, or enzymes. Absorption is the
intake of fluids or other substances by cells of the skin or mucous membranes.
4.3.6.4.3 Simple
Columnar Epithelium
4.3.6.4.3.1 The surface view
of simple columnar epithelium is similar to that of simple cuboidal tissue.
When sectioned at right angles to the surface, however, the cells appear
somewhat rectangular. The nuclei, usually located near the bases of the cells,
are commonly oval.
4.3.6.4.3.2 The luminal
surfaces (surfaces adjacent to the lumen, or cavity of a hollow organ, vessel,
or duct) of simple columnar epithelial cells are modified in several ways,
depending on location and function. Simple columnar epithelium lines the
gastrointestinal tract from the cardia of the stomach to the anus, the
gallbladder, and excretory ducts of many glands. In such sites, the cells
protect the underlying tissues. Many of the cells
are also modified to
4.3.6.4.3.3
4.3.6.4.3.4 START
4.3.6.4.3.5 Exhibit 4-1
Epithelial Tissues
4.3.6.4.3.5.1
4.3.6.4.4 Stratified
Cuboidal Epithelium
4.3.6.4.4.1
4.3.6.4.5 Stratified
Columnar Epithelium
4.3.6.4.5.1
4.3.6.4.6 Transitional
Epithelium
4.3.6.4.6.1
4.3.6.5
Pseudostratified Columnar Epithelium
4.3.6.6
4.3.7 Glandular Epithelium
4.3.7.1
4.3.7.2
Structural Classification of Exocrine
Glands
4.3.7.2.1
4.3.7.3
Functional Classification of Exocrine Glands
4.3.7.3.1
4.4 Connective Tissue
4.4.1 Introduction
4.4.1.1
This is the most abundant tissue in
the body and serves a binding and supporting function. With the exception of
cartilage, which is Avascular, connective tissue is highly vascular with a rich
blood supply. The cells of connective tissue are widely scattered as opposed to
closely packed and there is considerable intercellular substance (matrix). In
contrast to Epithelium, connective tissues do not occur on free surfaces, such
as the surfaces of a body cavity or the external surface of the body. The
general functions of connective tissues are protection, support, binding
together various organs, separating structures such as skeletal muscles, and
storage of reserve energy.
4.4.1.2
The tissue qualities are largely
determined by the intercellular substance in a connective tissue. This tissue
is a non-living substance and may consist of fluid, semi fluid, gel like, or
fibrous material.
4.4.1.3
The intercellular material found in
cartilage is firm but pliable. The intercellular material found in bone is
considerably harder and not pliable.
4.4.1.4
Fibroblast (FĪ-brō-blast)
4.4.1.4.1 A
large, flat cell that forms collagenous and elastic fibers and intercellular
substance of loose connective tissue
4.4.1.5
The intercellular substances are
produced by the cells of connective tissue (Fibroblasts).
4.4.1.6
These cells may also store fat, ingest
bacteria and cell debris, form anticoagulants, or give rise to antibodies that
protect against disease.
4.4.2 Classification
4.4.2.1.1 Connective
tissues may be classified in several ways. We will classify them as follows:
4.4.2.1.2 Embryonic connective tissue
4.4.2.1.2.1 Mesenchvme
4.4.2.1.2.2 Mucous connective
tissue
4.4.2.1.3
Adult connective
tissue
4.4.2.1.3.1 Connective tissue
proper
4.4.2.1.3.1.1 Loose
(areolar) connective tissue
4.4.2.1.3.1.2 Adipose
tissue
4.4.2.1.3.1.3 Dense
(collagenous) connective tissue
4.4.2.1.3.1.4 Elastic
connective tissue
4.4.2.1.3.1.5 Reticular connective tissue
4.4.2.1.3.2 Cartilage
4.4.2.1.3.2.1 Hyaline
cartilage
4.4.2.1.3.2.2
Fibrocartilage
4.4.2.1.3.2.3 Elastic
cartilage
4.4.2.1.3.3 Osseous (bone)
tissue
4.4.2.1.3.4 Vascular (blood)
tissue
4.4.2.1.4 Each
of the connective tissues described in the following sections is illustrated in
Exhibit 4-2.
4.4.2.1.5
4.4.3 Embryonic Connective Tissue
4.4.3.1
Connective tissue that is present
primarily in the. embryo or fetus is called embryonic connective tissue. The
term embryo refers to a developing human from fertilization through the
first two months of pregnancy; a fetus refers to a developing human from
the third month of pregnancy to birth.
4.4.3.2
One example of embryonic connective
tissue found almost exclusively in the embryo is mesenchyme (MEZen-kīm)-the
tissue from which all other connective tissues eventually arise. Mesenchyme is
located beneath the skin and along the developing bones of the embryo. Some
mesenchymal cells are scattered irregularly throughout adult connective tissue,
most frequently around blood vessels. Here mesenchymal cells differentiate into
fibroblasts that assist in wound healing.
4.4.3.3 Another kind of embryonic
connective tissue is mucous connective tissue (Wharton's jelly), found primarily in the fetus. This tissue is located in the umbilical
cord of the fetus, where it supports the wall of the cord.
4.4.4 Adult Connective Tissue
4.4.4.1
Adult connective tissue is
connective tissue that exists in the newborn that has differentiated from
mesenchyme and that does not change after birth. It is subdivided into several
kinds.
4.4.4.2 Exhibit 4-2 A
4.4.4.2.1 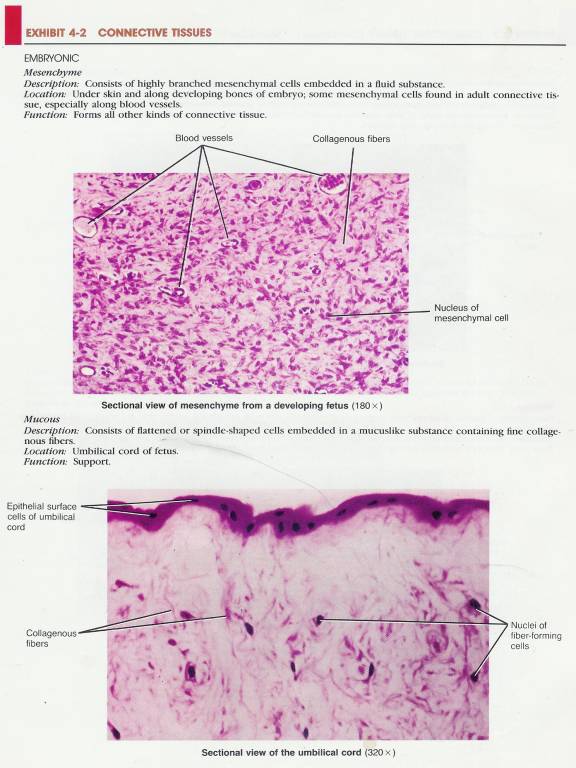
4.4.4.3 Exhibit 4-2 B
4.4.4.3.1 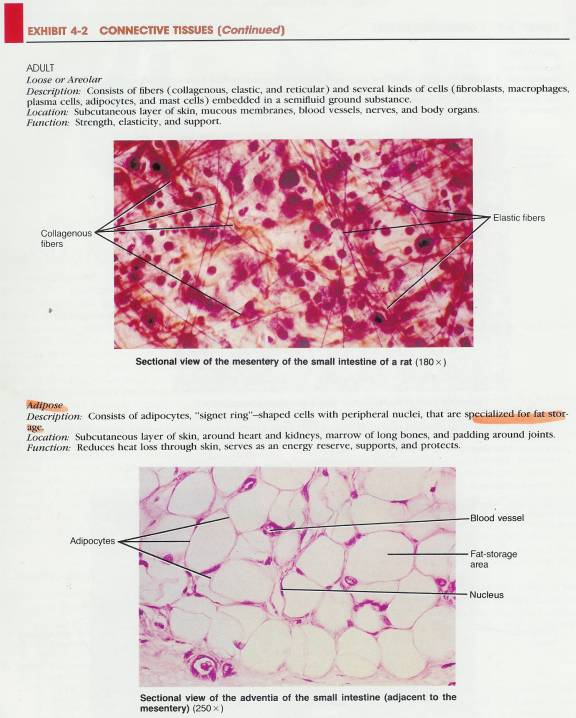
4.4.4.4 Exhibit 4-2 C
4.4.4.4.1 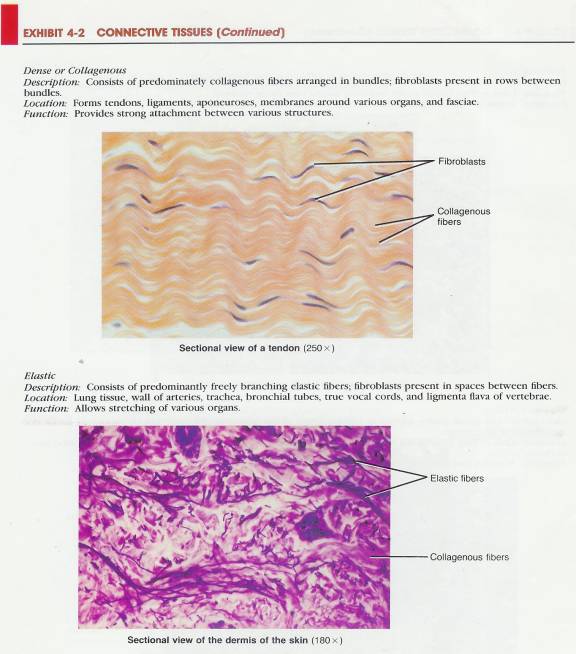
4.4.4.5 Exhibit 4-2 D
4.4.4.5.1 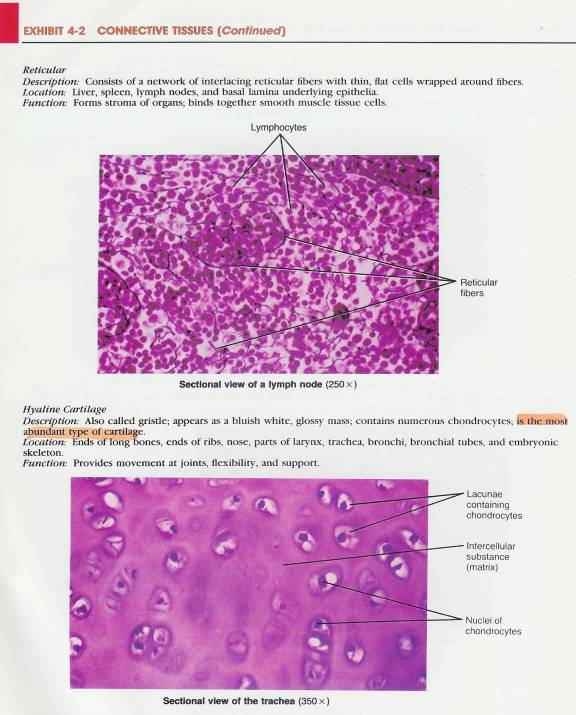
4.4.4.6 Exhibit 4-2 E
4.4.4.6.1 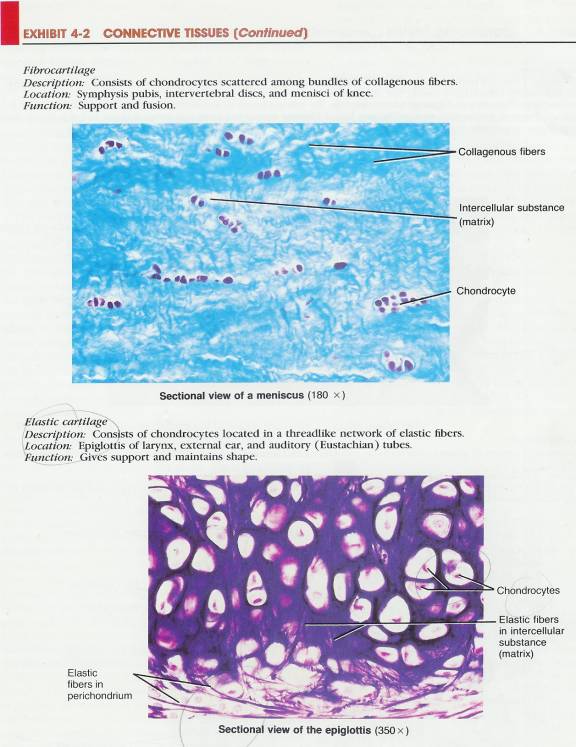
4.4.4.7 Exhibit 4-2 F
4.4.4.7.1 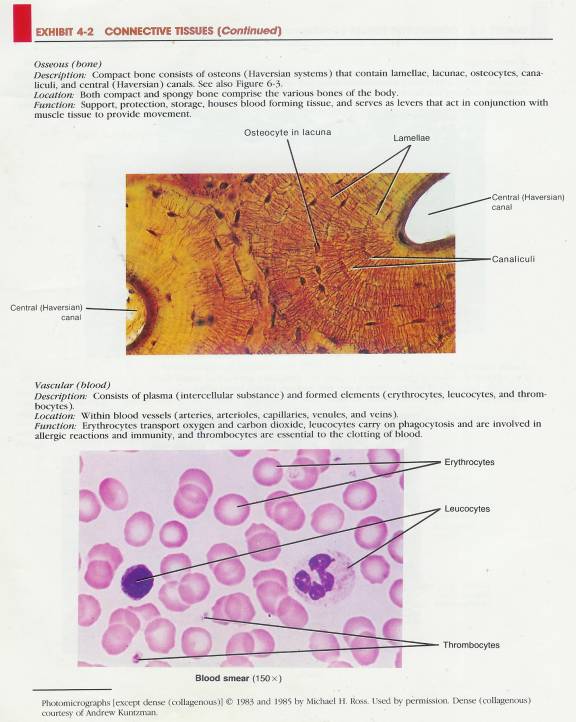
4.4.4.8 Connective Tissue Proper
4.4.4.8.1 There
are five examples of connective tissue that has more or less fluid
intercellular material and a fibroblast as the typical cell. All of this tissue
is called connective tissue proper.
4.4.4.8.2 Loose
(Areolar) Connective Tissue
4.4.4.8.2.1 Loose or areolar
(a-RĒ-ō-Iar) connective tissue is one of the most widely distributed
connective tissues in the body. Structurally, it consists of fibers and
several kinds of cells embedded in a semifluid intercellular substance. The
term loose refers to the loosely woven arrangement of fibers in the
intercellular substance. The fibers are neither abundant nor arranged to
prevent stretching.
4.4.4.8.2.2 The intercellular
substance consists primarily of hyaluronic acid, chondroitin sulfate, dermatan
sulfate, and keratan sulfate. It is secreted by connective tissue cells,
mostly fibroblasts. The intercellular substance normally facilitates the
passage of nutrients from the blood vessels of the connective tissue into
adjacent cells and tissues, although the thick consistency of this acid may
impede the movement of some drugs. But if an enzyme called hyaluronidase is
injected into the tissue, the intercellular substance changes to a watery
consistency. This feature is of clinical importance because the reduced
viscosity hastens the absorption and diffusion of injected drugs and fluids
through the tissue and thus can lessen tension and pain. Some bacteria, white
blood cells, and sperm cells produce hyaluronidase.
4.4.4.8.2.3 The three types of
fibers embedded between the cells of loose connective tissue are collagenous,
elastic, and reticular fibers. Collagenous fibers are very tough and
resistant to a pulling force, yet allow some flexibility in the tissue because
!hey are not taut. These fibers often occur in bundles. They are composed of
many minute fibers called fibrils lying parallel to one another. The bundle
arrangement affords a great deal of strength. Chemically, collagenous fibers
consist of the protein collagen. Elastic fibers, by contrast, are
smaller than collagenous fibers and freely branch and rejoin one another.
Elastic fibers consist of a protein called elastin. These fibers also provide
strength and have great elasticity, up to 50 percent of their length. Reticular
fibers also consist of collagen, plus some glycoprotein. They are very thin
fibers that form branching networks. Like collagenous fibers, reticular fibers
provide support and strength and also form the stroma
(framework) of many soft organs.
4.4.4.8.2.4
The cells in loose connective tissue are numerous and varied.
Most are fibroblasts-large, flat cells with branching processes. If the
tissue is injured, fibroblasts are believed to form collagenous fibers, elastic
fibers, and the intercellular substance. When a fibroblast becomes relatively
inactive, it is sometimes referred to as a fibrocyte.
.
4.4.4.8.2.5 Other cells found
in loose connective tissue are called fixed macrophages (MAK-r
ō-fā-jēz; macro = large; phagein = to. eat), or histiocytes,
which are derived from monocytes, a. type of
white blood cell. Macrophages are irregular in form with short branching projections
and are capable of engulfing bacteria and cellular debris by phagocytosis.
Thus, they provide a vital defense for the body.
4.4.4.8.2.6 A third kind of
cell in loose connective tissue is a plasma cell. It is small and
either round or irregular and develops from a type of white blood cell called a
lymphocyte (B cell). Plasma cells give rise to antibodies and,
accordingly, provide a defense mechanism through immunity. They are found in
many places in the body, but most are found in connective tissue, especially
that of the gastrointestinal tract and the mammary
glands.
4.4.4.8.2.7 Another cell in
loose connective tissue is a mast cell. The mast cell is found in
abundance along blood vessels. It forms heparin, an anticoagulant that prevents
blood from clotting in the vessels. Mast cells are also believed to produce
histamine and serotonin, chemicals that dilate small blood vessels.
4.4.4.8.2.8
Other cells in loose connective tissue include adipocytes
(fat cells) and leucocytes (white blood cells). .
4.4.4.8.2.9 Loose
connective tissue is continuous throughout the body. It is present in all
mucous membranes and around all blood vessels and nerves. And it occurs around
body organs and in the papillary (upper) region of the dermis of the skin.
Combined with adipose tissue, it forms the subcutaneous (sub'-kyoo-TĀ-nē-us;
sub = under; cut= skin) layer-the layer of tissue
that attaches the skin to underlying tissues and organs. The subcutaneous layer
is also referred to as the superficial fascia (FASH-ē-a) or hypodermis.
4.4.4.8.2.10
4.4.4.8.3 Clinical
Application
4.4.4.8.3.1 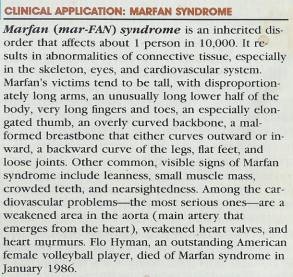
4.4.4.8.4 Adipose
Tissue
4.4.4.8.4.1 Adipose Tissue is
basically a form of loose connective tissue in which the cells, called adipocytes,
are specialized for fat storage. Adipocytes are derived from fibroblasts,
and the cells have the shape of a "signet ring" because the cytoplasm
and nucleus are pushed to the edge of the cell by a large droplet of fat.
Adipose tissue is found wherever loose connective tissue is located.
Specifically, it is in the subcutaneous layer below the skin, around the
kidneys, at the base and on the surface of the heart, in the marrow of long
bones, as padding around joints, and behind the eyeball in the orbit. Adipose
tissue is a poor conductor of heat and therefore reduces heat loss through the
skin. It is a major energy reserve and generally supports and protects various
organs.
4.4.4.8.4.2 Clinical
Application
4.4.4.8.4.2.1 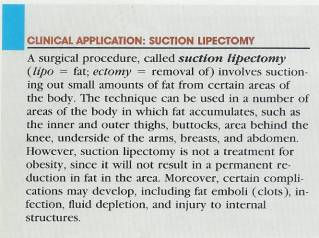
4.4.4.8.5 Dense
(Collagenous) Connective Tissue
4.4.4.8.5.1 Dense
(Collagenous) Connective Tissue has fibers that are closely packed than found
in loose connective tissue and the fibers can be irregularly or regularly
arranged. These fibers are made up of collagen.
4.4.4.8.5.2 Collagen
(KOL-a-jen
4.4.4.8.5.2.1 A
protein that is the main organic constituent of connective tissue
4.4.4.8.5.3 Irregularly
Arranged (fasciae)
4.4.4.8.5.3.1 When
the tensions on the connective tissue are exerted in various directions, the
fiber bundles are interwoven and without regular orientation. It forms most
fasciae, the reticular (deeper) region of the dermis of the skin, the
periosteum of bone, and the perichondrium of cartilage, and the membrane (fibrous)
capsules around organs, such as the kidneys, liver, testes, and lymph nodes.
4.4.4.8.5.4 Regularly Arranged
4.4.4.8.5.4.1 This
tissue is adapted for tension in one direction, and the fibers have an orderly,
parallel arrangement.
4.4.4.8.5.4.2 The
most common variety of dense regularly arranged connective tissue has a
predominance of collagenous fibers arranged in bundles.
4.4.4.8.5.4.3 Fibroblasts
are placed in rows between the bundles. The tissue is silvery white, tough, yet
somewhat pliable. Because of its great strength, it is the principal component
of tendons, which attach muscles to bones, aponeurosis
(ap’-ō-noo-RŌ-sēz), which are sheetlike tendons connecting one
muscle with another or with bone; and many ligaments (collagenous ligaments),
which hold bones together at joints.
4.4.4.8.5.5 Tissue Example
4.4.4.8.5.5.1 Plantar
Aponeurosis (PA) (A-paw-neurosis)
4.4.4.8.5.5.1.1
The plantar aponeurosis is Dense (Collagenous) Connective
Tissue, which is regularly arranged and stretches across the sole of the foot
similar to the palmar aponeurosis in the hand. It is a thickened layer of deep fascia,
which serves both a protective and supportive function to the underlying
muscles, vessels and nerves.
4.4.4.8.5.5.1.2
The fibers are oriented longitudinally and attached proximally
to the calcaneus extending over its surface. It is attached distally to the
metatarsophalangeal joints.
4.4.4.8.5.5.1.3
Functionally and histologically the plantar aponeurosis is
tendon like and acts as an extension of the Achilles tendon. During the push
off it is tightened along with the other plantar flexors, which pull on the
Achilles tendon whose fibers may interdigitate with the PA. As push off
progresses and the toes are extended the PA is further tightened. The tautness
of the PA helps maintain the arch during running and walking activities. During
stance phase the PA is less tight as intrinsic foot structures such as the long
and short plantar ligament assume the burden of arch support.
4.4.4.8.5.5.1.4
The aponeurosis divides distally into five digital slips, one
of which courses to each toe. Each division splits into two slips, which insert
on either side of the plantar ligament of the MP joint
4.4.4.8.5.5.1.5
Fibers extend from the margins of the aponeurosis to cover
partially both the medial and lateral plantar eminences.
4.4.4.8.5.5.1.6
The plantar aponeurosis and the long and short plantar
ligaments are the main structures that support the longitudinal arch of the
foot
4.4.4.8.5.5.2 Lateral
Cord of the Plantar Aponeurosis
4.4.4.8.5.5.2.1
Helps support the lateral longitudinal arch. It is attached to
the lateral Calcaneus blending with the plantar aponeurosis.
4.4.4.8.5.5.2.2
Distally this structure attaches to the base of the 5th
metatarsal bone.
4.4.4.8.5.5.3 Plantar
Fascia (fā-si-ă) (PF)
4.4.4.8.5.5.3.1
Extends over the medial and lateral side of the PA and covers
the abductor Hallucis (hǎ-li-cis) on the medial side and abductor Digiti
Minimi (dĭgĭtĭ mĭnĭmĭ) on the lateral side.
4.4.4.8.5.5.4 Long
Plantar Ligament (ligamentum plantare longum; long calcaneocuboid ligament;
superficial long plantar ligament) Illustration-Plantar
Illustration-Medial
4.4.4.8.5.5.4.1
The rough surface in front of the calcaneus processes gives
attachment to the long plantar ligament. On the distal end it attaches to the
cuboid bone whose plantar surface presents in front with a deep groove
(Peroneal Sulcus), which runs obliquely forward and medial ward (Peroneus
Longus tendon inserts). Behind this groove is a prominent ridge where the long
plantar ligament is attached.
4.4.4.8.5.5.4.2
The long plantar ligament is the longest of all the ligaments
of the tarsus: it is attached behind to the plantar surface of the calcaneus in
front of the tuberosity, and in front to the tuberosity on the plantar surface
of the cuboid bone, the more superficial fibers being continued forward to the
bases of the (sometimes second), third, fourth and fifth metatarsal bones. This
ligament converts the groove on the plantar surface of the cuboid into a canal
for the tendon of the Peroneus longus.
4.4.4.8.5.5.5 Plantar
Calcaneocuboid Ligament (Short Plantar Ligament) (ligamentum calcaneocuboideum
plantare; short calcaneocuboid ligament) Illustration-Plantar
Illustration-Medial
4.4.4.8.5.5.5.1
The plantar calcaneocuboid ligament lies nearer to the bones
than the preceding, from which it is separated by a little Areolar
(ă-realer) tissue. It is a short but wide band of great strength, and
extends from the tubercle and the depression in front of it, on the forepart of
the plantar surface of the calcaneus, to the plantar surface of the cuboid
behind the peroneal groove.
4.4.4.8.5.5.6 Plantar
Calcaneonavicular (Spring) Ligament
(ligamentum Calcaneonavicular plantare; inferior or internal
calcaneonavicular ligament; calcaneonavicular ligament) Illustration-Plantar
Illustration-Medial
4.4.4.8.5.5.6.1
The spring ligament passes from the anterior and medial
margins of the sustentaculum tali forward to the inferior and inferomedial
aspect of the Navicular. Its superior surface articulates with the underside of
the head of the talus. This ligament maintains apposition of the medial aspects
of the forefoot and Hindfoot and in so doing helps to maintain the normal
arched configuration of the foot. Laxity of the ligament allows a medial
separation between the calcaneus and forefoot, with the forefoot assuming an
abducted position with respect to the Hindfoot.
4.4.4.8.5.5.6.2
At the same time, the foot is allowed to “untwist” which
effectively lowers the normal arch of the foot, and the talar head is allowed
to move medially and inferiorly.
4.4.4.8.5.5.6.3
The plantar calcaneonavicular ligament is a broad and thick
band of fibers, which connects the anterior margin of the sustentaculum tali of
the calcaneus to the plantar surface of the Navicular. This ligament not only
serves to connect the calcaneus and Navicular, but supports the head of the
talus, forming part of the articular cavity in which it is received. The dorsal
surface of the ligament presents a fibrocartilaginous facet, lined by the
synovial membrane, and upon this a portion of the head of the talus rests. Its
plantar surface is supported by the tendon of the Tibialis posterior; its
medial border is blended with the forepart of the deltoid ligament of the
ankle-joint.
4.4.4.8.5.5.6.4
The plantar calcaneonavicular ligament, by supporting the head
of the talus, is principally concerned in maintaining the arch of the foot.
When it yields, the head of the talus is pressed downward, medialward, and
forward by the weight of the body, and the foot becomes flattened, expanded,
and turned lateralward, and exhibits the condition known as flat-foot. This
ligament contains a considerable amount of elastic fibers, so as to give
elasticity to the arch and spring to the foot; hence it is sometimes called the
“spring” ligament. It is supported, on its plantar surface, by the tendon of
the Tibialis posterior, which spreads out at its insertion into a number of
fasciculi, to be attached to most of the tarsal and metatarsal bones. This
prevents undue stretching of the ligament, and is a protection against the
occurrence of flat-foot; hence muscular weakness is, in most cases, the primary
cause of the deformity.
4.4.4.8.5.5.7
Retinaculum of foot/The Fascia Around the Ankle
4.4.4.8.5.5.7.1
Fibrous bands, or thickened portions of the fascia, bind down
the tendons in front of and behind the ankle in their passage to the foot. They
comprise three ligaments, viz., the transverse crural, the cruciate crural and
the laciniate; and the superior and inferior peroneal Retinacula.
4.4.4.8.5.5.7.2
Transverse Crural Ligament (ligamentum transversum cruris;
upper part of anterior annular ligament)
4.4.4.8.5.5.7.2.1
The transverse crural ligament binds down the tendons of
Extensor digitorum longus, Extensor hallucis longus, Peroneus Tertius, and
Tibialis anterior as they descend on the front of the tibia and fibula; under
it are found also the anterior tibial vessels and deep peroneal nerve. It is
attached laterally to the lower end of the fibula, and medially to the tibia;
above it is continuous with the fascia of the leg.
4.4.4.8.5.5.7.3
Cruciate Crural Ligament (ligamentum cruciatum cruris; lower
part of anterior annular ligament)
4.4.4.8.5.5.7.3.1
The cruciate crural ligament is a Y-shaped band placed in
front of the ankle-joint, the stem of the Y being attached laterally to the
upper surface of the calcaneus, in front of the depression for the interosseous
talocalcaneal ligament; it is directed medialward as a double layer, one lamina
passing in front of, and the other behind, the tendons of the Peroneus Tertius
and Extensor digitorum longus. At the medial border of the latter tendon these
two layers join together, forming a compartment in which the tendons are
enclosed. From the medial extremity of this sheath the two limbs of the Y
diverge: one is directed upward and medialward, to be attached to the tibial
malleolus, passing over the Extensor hallucis longus and the vessels and
nerves, but enclosing the Tibialis anterior by a splitting of its fibers. The
other limb extends downward and medialward, to be attached to the border of the
plantar aponeurosis, and passes over the tendons of the Extensor hallucis
longus and Tibialis anterior and also the vessels and nerves.
4.4.4.8.5.5.7.4
Laciniate Ligament (ligamentum laciniatum; internal annular
ligament)
4.4.4.8.5.5.7.4.1
The laciniate ligament is a strong fibrous band, extending
from the tibial malleolus above to the margin of the calcaneus below, converting
a series of bony grooves in this situation into canals for the passage of the
tendons of the Flexor muscles and the posterior tibial vessels and tibial nerve
into the sole of the foot. It is continuous by its upper border with the deep
fascia of the leg, and by its lower border with the plantar aponeurosis and the
fibers of origin of the Abductor hallucis muscle. Enumerated from the medial
side, the four canals which it forms transmit the tendon of the Tibialis
posterior; the tendon of the Flexor digitorum longus; the posterior tibial
vessels and tibial nerve, which run through a broad space beneath the ligament;
and lastly, in a canal formed partly by the talus, the tendon of the Flexor
hallucis longus.
4.4.4.8.5.5.7.5
Peroneal Retinacula (Superior and Inferior)
4.4.4.8.5.5.7.5.1
The peroneal Retinacula are fibrous bands, which bind down the
tendons of the Peronei longus and brevis as they run across the lateral side of
the ankle. The fibers of the superior retinaculum (external annular ligament)
are attached above to the lateral malleolus and below to the lateral surface of
the calcaneus. The fibers of the inferior retinaculum are continuous in front
with those of the cruciate crural ligament; behind they are attached to the
lateral surface of the calcaneus; some of the fibers are fixed to the peroneal
trochlea, forming a septum between the tendons of the Peronei longus and
brevis.
4.4.4.8.5.5.7.6
Mucous Sheaths of the Tendons Around the Ankle
4.4.4.8.5.5.7.6.1
All the tendons crossing the ankle-joint are enclosed for part
of their length in mucous sheaths, which have an almost uniform length of about
8 cm. each. On the front of the ankle (Fig. 441) the sheath for the Tibialis
anterior extends from the upper margin of the transverse crural ligament to the
interval between the diverging limbs of the cruciate ligament; those for the
Extensor digitorum longus and Extensor hallucis longus reach upward to just
above the level of the tips of the malleoli, the former being the higher. The
sheath of the Extensor hallucis longus is prolonged on to the base of the first
metatarsal bone, while that of the Extensor digitorum longus reaches only to
the level of the base of the fifth metatarsal. On the medial side of the ankle
(Fig. 442) the sheath for the Tibialis posterior extends highest up—to about 4
cm. above the tip of the malleolus—while below it stops just short of the
tuberosity of the navicular. The sheath for Flexor hallucis longus reaches up
to the level of the tip of the malleolus, while that for the Flexor digitorum
longus is slightly higher; the former is continued to the base of the first
metatarsal, but the latter stops opposite the first cuneiform bone. On the
lateral side of the ankle (Fig. 441) a sheath which is single for the greater
part of its extent encloses the Peronei longus and brevis. It extends upward for
about 4 cm. above the tip of the malleolus and downward and forward for about
the same distance.
4.4.4.8.6 Elastic
Connective Tissue
4.4.4.8.6.1 Unlike collagenous
connective tissue, elastic connective tissue has a predominance of freely
branching elastic fibers. These fibers give the unstained tissue a yellowish
color. Fibroblasts are present only in the spaces between the fibers. Elastic
connective tissue can be stretched and will snap back into shape. It is a
component of the walls of elastic arteries, trachea, bronchial tubes to the
lungs, and the lungs themselves. Elastic connective tissue provides stretch
and strength, allowing structures to perform their functions efficiently.
Yellow elastic ligaments, as contrasted with collagenous ligaments, are
composed mostly of elastic fibers; they form the ligamenta flava of the
vertebrae (ligaments between successive vertebrae), the suspensory ligament of
the penis, and the true vocal cords.
4.4.4.8.7 Reticular
Connective Tissue
4.4.4.8.7.1 Reticular
connective tissue consists of interlacing reticular fibers. It helps to form a
delicate supporting stroma (framework) for many organs, including the liver,
spleen, and lymph nodes. It is also found in the basal lamina underlying
epithelia and around blood vessels and muscle. Reticular connective tissue also
helps to bind together the fibers (cells) of smooth muscle tissue.
4.4.4.9 Cartilage
4.4.4.9.1 Cartilage
is capable of enduring considerably more stress than the tissues just
discussed. Unlike other connective tissues, cartilage has no blood vessels or
nerves, except for those in the perichondrium (membranous covering). Cartilage
consists of a dense network of collagenous fibers and elastic fibers firmly
embedded in chondroitin sulfate, a jellylike intercellular substance (matrix).
Whereas the strength of cartilage is due to its collagenous fibers, its
resilience (ability to assume its original shape after deformation) is due to
chondroitin sulfate. The cells of mature cartilage, called chondrocytes (KON-drō-sīts),
occur singly or in groups within spaces called lacunae ( la-KOO-nē) in the intercellular substance. The
surface of cartilage is surrounded by irregularly arranged dense. connective
tissue called the perichondrium (per'-i-KON-drē-um; peri = around;
chondro = cartilage). Three kinds of cartilage are recognized: hyaline
cartilage, fibrocartilage, and elastic cartilage (Exhibit 4-2).
4.4.4.9.2 Hyaline
Cartilage
4.4.4.9.2.1 This cartilage,
also called gristle, 'appears in the body as a bluish-white, shiny
substance. The collagenous fibers, although present, are not visible with
ordinary staining techniques, and the prominent chondrocytes are found in
lacunae. .Hyaline cartilage is the most abundant kind of cartilage in the body.
It is found at joints over the ends of the long bones (where it is called articular
cartilage) and forms the costal cartilages at the ventral
ends of the ribs. Hyaline cartilage also helps to form the nose, larynx,
trachea, bronchi, and bronchial tubes leading to the lungs. Most of the
embryonic skeleton consists of hyaline cartilage. Hyaline cartilage affords flexibility
and support and, as articular cartilage, reduces friction and absorbs shock.
4.4.4.9.3 Fibrocartilage
4.4.4.9.3.1 Chondrocytes
scattered through many bundles of visible collagenous fibers are found in this
type of cartilage. Fibrocartilage is found at the symphysis pubis, the point
where the coxal (hip) bones fuse anteriorly at the midline. It is
also found in the intervertebral discs between vertebrae and the menisci of
the knee. This tissue combines strength and rigidity.
4.4.4.9.4 Elastic
Cartilage
4.4.4.9.4.1 In this tissue,
chondrocytes are located in a threadlike network of elastic fibers. Elastic
cartilage provides strength and elasticity and maintains the shape of certain
organs-the epiglottis of the larynx, the external part of the ear (pinna), and
the auditory (Eustachian) tubes.
4.4.4.9.5 Growth
of Cartilage
4.4.4.9.5.1 The growth of
cartilage follows two basic patterns. In. interstitial (endogenous)
growth, the cartilage increases rapidly in size through the
division of existing chondrocytes and continuous deposition of increasing
amounts of intercellular matrix by the chondrocytes. The formation of new
chondrocytes and their production of new intercellular matrix causes the
cartilage to expand from within-thus, the term interstitial growth. This
growth pattern occurs while the cartilage is young and pliable-during childhood
and adolescence.
4.4.4.9.5.2 In appositional (exogenous) growth, the
growth of cartilage occurs because of the activity of the inner chondrogenic
layer of the perichondrium. The deeper cells of the perichondrium, the
fibroblasts, divide. Some differentiate into chondroblasts (immature cells that
develop into specialized cells) and then into chondrocytes. As differentiation
occurs, the chondroblasts become surrounded with intercellular matrix and
become chondrocytes. As a result, the matrix is deposited on the surface of
the cartilage, increasing its size. The new layer of cartilage is added
beneath the perichondrium on the surface of the cartilage, causing it to grow
in width. Appositional growth starts later than interstitial growth and
continues throughout life.
4.4.4.10 Osseous Tissue (Bone)
4.4.4.10.1
Together, cartilage, joints, and osseous (OS-ē-us) tissue (bone) comprise the skeletal
system. Mature bone cells are called osteocytes.
The intercellular substance consists of mineral salts, primarily
calcium phosphate and calcium carbonate, and collagenous fibers. The salts are
responsible for the hardness of bone.
4.4.4.10.2
Bone tissue is classified as either compact (dense) or
spongy (cancellous), depending on how the intercellular substance and cells are
organized. At this point, we will discuss compact bone only. The basic unit of
compact bone is called an osteon
(Haversian system). Each osteon consists of lamellae, concentric rings of hard,
intercellular substance; lacunae,
small spaces between lamellae that contain osteocytes; canaliculi, radiating minute canals
that provide numerous routes so that nutrients can reach osteocytes and wastes
can be removed from them; and a central
(Haversian) canal that contains blood vessels and nerves. Notice that
whereas osseous tissue is vascular, cartilage is not. Also, the lacunae of
osseous tissue are interconnected by canaliculi; those of cartilage are not.
4.4.4.10.3
Functionally, the skeletal system supports soft
tissues, protects delicate structures, works with skeletal muscles to
facilitate movement, stores calcium and phosphorus, houses red marrow, which
produces several kinds of blood cells, and houses yellow marrow, which contains
lipids as an energy source.
4.4.4.10.4
The details of compact and spongy bone are discussed in
Chapter 6.
4.4.4.11 Vascular Tissue (Blood)
4.4.4.11.1
Vascular tissue (blood) is a liquid connective tissue that
consists of an intercellular substance called plasma and formed elements (cells
and cell-like structures).
4.4.4.11.2
Plasma is a straw-colored liquid that consists mostly of water
plus some dissolved substances (nutrients, enzymes, hormones, respiratory
gases, and ions). The formed elements are erythrocytes (red blood cells),
leucocytes (white blood cells), and thrombocytes (platelets). The fibers
characteristic of all connective tissues are present in blood only when it is
clotted.
4.4.4.11.3
Erythrocytes function in transporting oxygen to body cells and
removing carbon dioxide from them. Leucocytes
are involved in phagocytosis, immunity, and allergic reactions. Thrombocytes function in blood
clotting.
4.4.4.11.4
The details of blood are considered in Chapter 19.
4.5 Membranes
4.5.1
The combination of an epithelial layer
and an underlying connective tissue layer constitutes an epithelial membrane. The
principal, epithelial membranes of the body are mucous membranes, serous
membranes, and the cutaneous membrane or skin.
Another kind of membrane, a synovial membrane, does not contain epithelium.
4.5.2 Mucous Membranes
4.5.2.1 A mucous membrane, or
mucosa, lines a body cavity that opens
directly to the exterior. Mucous membranes line the entire gastrointestinal,
respiratory, excretory, and reproductive tracts (see Figure.24- 2) and consist
of a lining layer of epithelium and an underlying layer of connective tissue.
In addition, most mucous membranes also contain a layer of smooth muscle called
the muscularis mucosae.
4.5.2.2
The epithelial layer of a mucous
membrane secretes mucus, which prevents the cavities from drying out. It also
traps particles in the respiratory passageways and lubricates food as it moves
through the gastrointestinal tract. In addition, the epithelial layer is
responsible for the secretion of digestive enzymes and the absorption of food.
4.5.2.3
The connective tissue layer of a mucous
membrane is called the lamina propria. The lamina propria is so named because it belongs to the mucous
membrane (proprius = one's own). It binds the epithelium to the underlying
structures and allows some flexibility of the membrane. It also holds the blood
vessels in place, protects underlying muscles from abrasion or puncture, and
allows the diffusion of oxygen and nutrients to the epithelium covering it, and
the diffusion of carbon dioxide and wastes from the epithelium covering it.
4.5.2.4 The muscularis mucosae contains
smooth muscle fibers and separates the mucosa from the submucosa beneath. Its
role in the gastrointestinal tract is considered in Chapter 24.
4.5.3 Serous Membranes
4.5.3.1 A serous membrane, or
serosa, lines a body cavity that does not
open directly to the exterior, and it covers the organs that lie within the
cavity. Serous membranes consist of thin layers of loose connective tissue
covered by a layer of mesothelium, and they are composed of two portions. The
part attached to the cavity wall is called the parietal (pa-RĪ-e-tal) portion. The part that covers and
attaches to the organs inside these cavities is the visceral portion. The serous
membrane lining the thoracic cavity and covering the lungs is called the pleura (see Figure 1-7). The
membrane lining the heart cavity and covering the heart is the pericardium (cardio = heart): The serous membrane lining the abdominal cavity and covering
the abdominal organs and some pelvic organs is called the peritoneum.
4.5.3.2
The epithelial layer of a serous
membrane secretes a lubricating fluid, called serous fluid, that allows the organs
to glide easily against one another or against the walls of the cavities. The
connective tissue layer of the serous membrane consists of a relatively thin
layer of loose connective tissue.
4.5.4 Cutaneous Membrane
4.5.4.1
The cutaneous membrane, or skin,
constitutes an organ of the integumentary system and is discussed in the next
chapter.
4.5.5 Synovial Membranes
4.5.5.1
Synovial membranes line the cavities
of the freely movable joints (see Figure 9-1a). Like serous membranes, they
line structures that do not open to the exterior. Unlike mucous, serous, and
cutaneous membranes, they do not contain epithelium and are therefore not
epithelial membranes. They are composed of loose connective tissue with
elastic fibers and varying amounts of fat. Synovial membranes secrete synovial fluid, which lubricates the
articular cartilage at the ends of bones as they move at joints and nourishes
the articular cartilage covering the bones that form the joints. These are articular
synovial membranes. Other synovial membranes line cushioning sacs, called
bursae, and tendon sheaths in our hands and feet that facilitate the movement
of muscle tendons.
4.6 Muscle Tissue
4.6.1 Muscle tissue consists of fibers (cells) that are highly specialized for the
active generation of force for contraction. As a result of this
characteristic, muscle tissue provides motion, maintenance of posture, and heat
production. On the basis of certain structural and functional characteristics,
muscle tissue is classified into three types: skeletal, cardiac, and smooth
(Exhibit 4-3).
4.6.2 Skeletal muscle tissue is named for its location attached to bones. It is also striated; that is, the fibers
(cells) contain alternating light and dark bands (striations) that are
perpendicular to the long axes of the fibers. The striations are visible under
a microscope. Skeletal muscle tissue is also voluntary because it can be made to
contract or relax by conscious control. A single skeletal muscle fiber is
cylindrical, and the fibers are parallel to each other in a tissue. Each muscle
fiber contains a plasma membrane, the sarcolemma,
surrounding the cytoplasm, or sarcoplasm. Skeletal muscle fibers
are multinucleate (they have more than one nucleus), and the nuclei lie close
to the sarcolemma. The contractile elements of skeletal muscle fibers are
proteins called myofilaments. They contain wide, transverse, dark bands and narrow light ones
that give the fibers the striated appearance.
4.6.3 Cardiac muscle tissue forms the bulk of the wall of the heart. Like skeletal muscle
tissue, it is striated. However, unlike skeletal muscle tissue, it is usually
involuntary; its contraction is usually not under conscious control. Cardiac
muscle fibers are roughly quadrangular and branch to form networks throughout
the tissue. The fibers usually have only one nucleus that is centrally located.
Cardiac muscle fibers are bound to each other by transverse thickenings of the
sarcolemma called intercalated discs. These are unique to cardiac muscle and serve to strengthen the
tissue and aid muscle action potential conduction by way of channels called gap junctions.
4.6.4 Smooth muscle tissue is located in the walls of hollow internal structures such as blood
vessels, the stomach, intestines, and urinary bladder. Smooth muscle fibers are
usually involuntary, and they are nonstriated (smooth). Each smooth muscle fiber is thickest in the midregion, with either
end tapering to a point, and contains a single, centrally located nucleus.
4.6.5 A more detailed discussion of muscle tissue is considered in
Chapter 10.
4.6.6 Exhibit 4-3
4.6.6.1
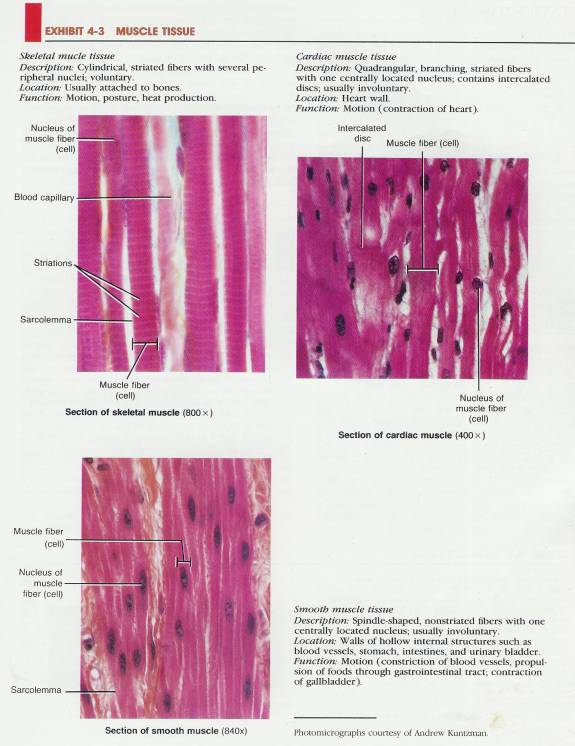
4.7 Nervous Tissue
4.7.1 Despite the tremendous complexity of the nervous system, it consists
of only two principal kinds of cells: neurons and neuroglia. Neurons, or nerve cells, are highly
specialized cells that are sensitive to various stimuli; converting stimuli
to nerve impulses; and conducting nerve impulses to other neurons, muscle
fibers, or glands. Neurons are the structural and functional units of the
nervous system. Most consist of three basic portions: cell body and two kinds
of processes called dendrites and axons (Exhibit 4-4). The cell body (perikaryon) contains the
nucleus and other organelles. Dendrites are highly branched processes of the cell body that conduct nerve
impulses toward the cell body. Axons are single, long processes of the cell body that conduct nerve
impulses away from the cell body.
4.7.2 Neuroglia are cells that protect and support neurons. They are of clinical
interest because they are frequently the sites of tumors of the nervous system.
4.7.3 The detailed structure and function of neurons and neuroglia are
considered in Chapter 12.
4.8 Tissue Repair: An Attempt to Restore Homeostasis
4.8.1 Tissue repair is the process by which new tissues replace dead or damaged cells. New
cells originate by cell duplication from the stroma, the supporting connective
tissue, or from the parenchyma, cells that form the organ's functioning part. The epithelial cells
that secrete and absorb are the parenchymal cells of the intestine, for example.
The restoration of an injured organ or tissue to normal structure and function
depends entirely on which type of cell-parenchymal or stromal-is active in the
repair. If only parenchymal elements accomplish the repair, a perfect or near
-perfect reconstruction of the injured tissue may occur. However, if the
fibroblast cells of the stroma are active in the repair, the tissue will be
replaced with new connective tissue called scar tissue. In this process,
fibroblasts synthesize collagen and protein polysaccharides that aggregate to
form scar tissue. The process of scar tissue formation is known as fibrosis. Since scar tissue is not
specialized to perform the functions of the parenchymal tissue, the overall
function of the tissue is impaired. If the rate of collagen breakdown in a scar
exceeds production, the scar becomes softer and less bulky. If, on the other
hand, the rate of collagen production exceeds breakdown, a keloid (hypertrophic) scar develops.
Such a scar is sharply elevated, irregularly shaped, and unsightly.
4.8.2 Clinical Application
4.8.2.1
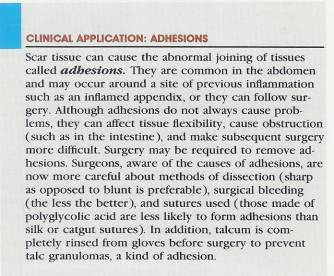
4.8.3 Exhibit 4-4
4.8.3.1
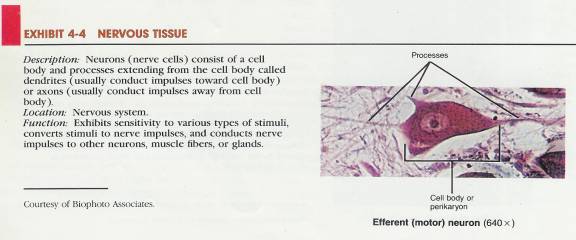
4.8.4 The cardinal factor in tissue
repair-lies in the capacity of parenchymal tissue to regenerate. This capacity,
in turn, depends on the ability of the parenchymal cells to replicate quickly.
4.8.5 Repair Process
4.8.5.1 If injury to a tissue is slight, repair may sometimes be
accomplished with the drainage and reabsorption of pus (accumulation of
leucocytes and fluid resulting from inflammation), followed by parenchymal
regeneration. When the area of skin loss is great, fluid moves out of the
capillaries and the area becomes dry. Fibrin, an insoluble protein in a blood
clot, seals the open tissue by hardening into a scab.
4.8.5.2
When tissue and cell damage are
extensive and severe, as in large, open wounds, both the connective tissue
stroma and the parenchymal cells are active in repair. This repair involves the
rapid cell division of many fibroblasts, the manufacture of new collagenous
fibers to provide strength, and an increase by cell division of the number of
small blood vessels in the area. All these processes create an actively growing
connective tissue called granulation tissue. This new granulation tissue forms across a wound or surgical
incision to provide a framework (stroma). The framework supports the epithelial
cells that migrate into the open area and fill it. The newly formed granulation
tissue also secretes a fluid that kills bacteria.
4.8.6 Conditions Affecting Repair
4.8.6.1
Three factors affect tissue repair:
nutrition, blood circulation, and age. Nutrition is vital in the healing
process since a great demand is placed on the body's store of nutrients.
Protein-rich diets are important since most of the cell structure is made from
proteins. Vitamins also play a direct role in wound healing. Among the vitamins
involved and their roles in wound healing are the following:
4.8.6.1.1
Vitamin A is essential in the replacement of
epithelial tissues, especially in the respiratory tract.
4.8.6.1.2 The B
vitamins-thiamine, nicotinic acid, riboflavin-are coenzymes needed by many
enzyme systems in cells. They are needed especially for the enzymes involved in
decomposing glucose to CO2 and H2O, which is crucial to
both heart and nervous tissue. These vitamins may relieve pain in some cases
and are necessary for division of the cells that accomplish repair.
4.8.6.1.3 Vitamin
C directly affects the normal production and maintenance of intercellular
substances. It is required for the manufacture of cementing elements of
connective tissues, especially collagen. Vitamin C also strengthens and
promotes the formation of new blood vessels. With vitamin C deficiency, even
superficial wounds fail to heal, and the walls of the blood vessels become
fragile and are easily ruptured.
4.8.6.1.4 Vitamin
D is necessary for the proper absorption of calcium from the intestine. Calcium
gives bones their hardness and is necessary for the healing of fractures.
4.8.6.1.5 Vitamin
E is believed to promote healing of injured tissues and may prevent scarring.
4.8.6.1.6 Vitamin
K assists in the clotting of blood and thus prevents the injured person from
bleeding to death. Although vitamin K is essential for the formation of certain
clotting factors, it is not, itself, a coagulant. The body, with the aid of
colon bacteria, normally synthesizes its own vitamin K. In humans, bacteria in
the colon synthesize vitamin K which can be' absorbed and utilized.
4.8.6.2
In
tissue repair, proper blood circulation is indispensable. It is the blood that
transports oxygen, nutrients, antibodies, and many defensive cells to the site
of injury. The blood also plays an important role in the removal of tissue
fluid, blood cells that have been depleted of oxygen, bacteria, foreign bodies,
and debris. These elements would otherwise interfere with healing.
4.8.6.3
Generally,
tissues heal faster and leave less obvious scars in the young than in the aged.
The young body is generally in a much better nutritional state, its tissues
have a better blood supply, and the cells of younger people have a faster
metabolic rate. Thus, cells can duplicate their materials and divide more
quickly.
4.8.7 Summary
4.8.7.1.1 1. A
tissue is a group of similar cells and their intercellular substance that have
a similar embryological origin and are specialized for a particular function.
4.8.7.1.2 2.
Depending on their function and structure, the various tissues of the body are
classified into four principal types: epithelial, connective, muscular, and
nervous.
4.8.7.2.1 1.
Epithelium has many cells, little intercellular material, and no blood vessels
(avascular). It is attached to connective tissue by a basement membrane. It
can replace itself.
4.8.7.2.2 2. The
subtypes of epithelium include covering and lining epithelium and glandular
epithelium.
4.8.7.3 Covering and Lining Epithelium (p.
93)
4.8.7.3.1 1. Layers
are arranged as simple (one layer), stratified (several layers), and
pseudostratified (one layer that appears as several); cell shapes include
squamous (flat), cuboidal (cubelike), columnar (rectangular), and transitional
(variable).
4.8.7.3.2 2.
Simple squamous epithelium is adapted for diffusion and filtration
and is found in lungs and kidneys. Endothelium lines the heart and blood
vessels. Mesothelium lines the thoracic and abdominopelvic cavities and covers
the organs within' them.
4.8.7.3.3 3.
Simple cuboidal epithelium is adapted for secretion and absorption. It is found
covering ovaries, in kidneys and eyes, and lining some glandular ducts.
4.8.7.3.4 4.
Nonciliated simple columnar epithelium lines most of the gastrointestinal
tract. Specialized cells containing microvilli perform absorption. Goblet cells
secrete mucus. In a few portions of the respiratory tract, the cells are
ciliated to move foreign particles trapped in mucus out of the body.
4.8.7.3.5 5.
Stratified squamous epithelium is protective. It lines the upper gastrointestinal
tract and vagina and forms the outer layer of skin.
4.8.7.3.6 Stratified
cuboidal epithelium is found in adult sweat glands, pharynx, epiglottis, and
portions of the urethra.
4.8.7.3.7 7.
Stratified columnar epithelium protects and secretes. It is found in the male
urethra and large excretory ducts.
4.8.7.3.8 8.
Transitional epithelium lines the urinary bladder and is capable of
stretching.
4.8.7.3.9 9.
Pseudostratified columnar epithelium has only one layer but gives the
appearance of many. It lines larger excretory ducts, parts of urethra, auditory
(Eustachian) tubes, and most upper respiratory structures, where it protects
and secretes.
4.8.7.4
Glandular
Epithelium (p. 100)
4.8.7.4.1 A
gland is a single cell or a mass of epithelial cells adapted for secretion.
4.8.7.4.2 Exocrine
glands (sweat, oil, and digestive glands) secrete into ducts or directly onto a
free surface.
4.8.7.4.3 Structural classification includes
unicellular and multicellular glands; multicellular glands are further
classified as tubular, acinar, tubuloacinar, simple, and compound.
4.8.7.4.4 Functional classification includes
holocrine, merocrine, and apocrine glands.
4.8.7.4.5 Endocrine glands secrete hormones into
the blood.
4.8.7.5
Connective
Tissue (p. 102)
4.8.7.5.1 Connective
tissue is the most abundant body tissue. It has few cells, an extensive
intercellular substance, and a rich blood supply (vascular), except for
cartilage. It does not occur on free surfaces.
4.8.7.5.2 2. The
intercellular substance determines the tissue's qualities.
4.8.7.5.3 Connective
tissue protects, supports, and binds organs together.
4.8.7.5.4 4.
Connective tissue is classified into two principal types: embryonic and adult.
4.8.7.5.5 Embryonic Connective Tissue (p. 102) .
4.8.7.5.5.1 Mesenchyme forms
all other connective tissues.
4.8.7.5.5.2 Mucous connective
tissue is found in the umbilical cord of the fetus, where it gives support.
4.8.7.5.6 Adult Connective Tissue (p. 102)
4.8.7.5.6.1 Adult connective
tissue is connective tissue that differentiates from mesenchyme and exists in
the newborn and does not change after birth. It is subdivided into several
kinds: connective tissue proper, cartilage, bone tissue, and vascular tissue.
4.8.7.5.6.2 Connective tissue
proper has a more or less fluid intercellular material, and a typical cell is
the fibroblast. Five examples of such tissues may be distinguished.
4.8.7.5.6.3 Loose (areolar)
connective tissue is one of the most widely distributed connective tissues in the
body. Its intercellular substance (hyaluronic acid) contains fibers
(collagenous, elastic, and reticular) and various cells (fibroblasts, macrophages,
plasma, and mast). Loose connective tissue is found in all mucous membranes,
around body organs, and in the subcutaneous layer.
4.8.7.5.6.4 Adipose tissue is
a form of loose connective tissue in which the cells, called adipocytes, are
specialized for fat storage. It is found in the subcutaneous layer and around
various organs.
4.8.7.5.6.5 Dense
(collagenous) connective tissue has a close packing of fibers (regularly or
irregularly arranged). It is found as a component of fascia, membranes of
organs, tendons, ligaments, and aponeuroses.
4.8.7.5.6.6 Elastic connective
tissue has a predominance of freely branching elastic fibers that give it a
yellow color. It is found in elastic arteries, trachea, bronchial tubes, and
true vocal cords.
4.8.7.5.6.7 Reticular
connective tissue consists of interlacing reticular fibers and forms the stroma
of the liver, spleen, and lymph nodes.
4.8.7.5.6.8
8. Cartilage has a jellylike matrix containing
collagenous and " elastic fibers and chondrocytes.
4.8.7.5.6.9 Hyaline cartilage
is found in the embryonic skeleton, at the ends of bones, in the nose, and in
respiratory structures. It is flexible, allows movement, and provides support.
4.8.7.5.6.10
Fibrocartilage connects the pelvic bones and the vertebrae. It
provides strength.
4.8.7.5.6.11
Elastic cartilage maintains the shape of organs such as the
larynx, auditory (Eustachian) tubes, and external ear.
4.8.7.5.6.12
The growth of cartilage is accomplished by interstitial growth
(from within) and appositional growth (from without).
4.8.7.5.6.13
Osseous tissue (bone) consists of mineral salts and collagenous
fibers that contribute to the hardness of bone and cells called osteocytes. It
supports, protects, helps provide movement, stores minerals, and houses red
marrow.
4.8.7.5.6.14
Vascular tissue (blood) consists of plasma and formed elements
(erythrocytes, leucocytes, and thrombocytes). Functionally, its cells
transport, carry on phagocytosis, participate in allergic reactions, provide
immunity, and bring about blood clotting.
4.8.7.6
Membranes
(p.111)
4.8.7.6.1 1. An
epithelial membrane is an epithelial layer overlying a connective tissue layer.
Examples are mucous, serous, and cutaneous membranes.
4.8.7.6.2 2.
Mucous membranes line cavities that open to the exterior, such as the
gastrointestinal tract.
4.8.7.6.3 Serous
membranes (pleura, pericardium, peritoneum) line closed cavities and cover the
organs in the cavities. These membranes consist of parietal and visceral
portions.
4.8.7.6.4 The
cutaneous membrane is the skin.
4.8.7.6.5 Synovial
membranes line joint cavities, bursae, and tendon sheaths
and do not contain epithelium.
4.8.7.7
Muscle
Tissue (p. 112)
4.8.7.7.1 1.
Muscle tissue is modified for contraction and thus provides motion, maintenance of posture, and
heat production,
4.8.7.7.2 2,
Skeletal muscle tissue is attached to bones, is striated, and is voluntary,
4.8.7.7.3 3.
Cardiac muscle tissue forms most of the heart wall, is striated, and is usually
involuntary.
4.8.7.7.4 Smooth muscle tissue is found in the
walls of hollow internal structures (blood vessels and viscera), is
nonstriated, and is usually involuntary.
4.8.7.8
Nervous
Tissue (p. 114)
4.8.7.8.1 The nervous system is composed of
neurons (nerve cells) and neuroglia (protective and supporting cells).
4.8.7.8.2 2.
Most neurons consist of a cell body and two types of processes called
dendrites and axons.
4.8.7.8.3 3.
Neurons are sensitive to stimuli, convert stimuli into nerve impulses,
and conduct nerve impulses.
4.8.7.9
Tissue Repair: An Attempt to Restore Homeostasis (p. 114)
4.8.7.9.1 1.
Tissue repair is the replacement of damaged or destroyed cells by healthy ones.
4.8.7.9.2 It begins during the active phase of
inflammation and is not completed until after harmful substances in the
inflamed area have been neutralized or removed.
4.8.7.9.3 Repair
Process (p. 114)
4.8.7.9.3.1 If the injury is superficial, tissue
repair involves pus removal (if pus is present), scab formation, and
parenchymal regeneration.
4.8.7.9.3.2 If damage is
extensive, granulation tissue is involved.
4.8.7.9.4 Conditions
Affecting Repair (p. 115)
4.8.7.9.4.1 Nutrition is important to tissue
repair. Various vitamins (A, some B, D, C, E, and K) and a protein-rich diet
are needed.
4.8.7.9.4.2 Adequate
circulation of blood is needed.
4.8.7.9.4.3 The tissues of
young people repair rapidly and efficiently; the process slows down with aging.
5.1
Skin
5.1.1
Physiology
5.1.2
Structure
5.1.2.1
Epidermis
5.1.2.1.1
Stratum
Basale
5.1.2.1.2
Stratum
Spinosum
5.1.2.1.3
Stratum Granulosum
5.1.2.1.4
Stratum
Lucidum
5.1.2.1.5
Stratum
Comeum
5.1.2.2
Dermis
5.1.2.3
Skin
Color
5.1.2.4
Epidermal
Ridges and Grooves
5.1.3
Skin
Wound Healing: Restoration of Homeostasis
5.1.3.1
Epidermal
Wound Healing
5.1.3.2
Deep
Wound Healing
5.1.4
Epidermal
Derivatives
5.1.4.1
Hair
5.1.4.2
Glands
5.1.4.2.1
Sebaceous
(Oil) Glands
5.1.4.2.2
Sudoriferous (Sweat)
Glands
5.1.4.2.3
Ceruminous
Glands
5.1.5
Homeostasis
of Body Temperature
5.1.6
Aging
and The Integumentary System
5.1.7
Developmental
Anatomy of the Integumentary System
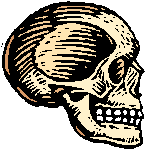
7.1
Axial
Skeleton
7.1.1
Types
of Bones
7.1.2
Surface Markings
Back Table
of Contents References
7.1.2.1.1
7.1.2.2.1
Fissure
(FISH-ur)
7.1.2.2.1.1 Description
7.1.2.2.1.1.1
7.1.2.2.1.2 Example
7.1.2.2.1.2.1
7.1.2.2.2
Foramen
(fō-RĀ-men; foramen=hole)
7.1.2.2.2.1 Description
7.1.2.2.2.1.1
7.1.2.2.2.2 Example
7.1.2.2.2.2.1
7.1.2.2.3
Meatus
(mē-Ā-tus; meatus=canal)
7.1.2.2.3.1 Description
7.1.2.2.3.1.1
7.1.2.2.3.2 Example
7.1.2.2.3.2.1
7.1.2.2.4
Paranasal
Sinus (sin=cavity)
7.1.2.2.4.1 Description
7.1.2.2.4.1.1
7.1.2.2.4.2 Example
7.1.2.2.4.2.1
7.1.2.2.5
Groove or
Sulcus (Sulcus=ditchlike groove)
7.1.2.2.5.1 Description
7.1.2.2.5.1.1
7.1.2.2.5.2 Example
7.1.2.2.5.2.1
7.1.2.2.6
Fossa
(fossa=basinlike depression)
7.1.2.2.6.1 Description
7.1.2.2.6.1.1
7.1.2.2.6.2 Example
7.1.2.2.6.2.1
Back Table
of Contents References
7.1.2.3.1
Condyle
(KON-dīl; condylus=knucklelike process)
7.1.2.3.1.1 Description
7.1.2.3.1.1.1
7.1.2.3.1.2 Example
7.1.2.3.1.2.1
7.1.2.3.2
Head
7.1.2.3.2.1 Description
7.1.2.3.2.1.1
7.1.2.3.2.2 Example
7.1.2.3.2.2.1
7.1.2.3.3
Facet
7.1.2.3.3.1 Description
7.1.2.3.3.1.1
7.1.2.3.3.2 Example
7.1.2.3.3.2.1
7.1.2.3.4
Tubercle
(TOO-ber-kul; tube=knob)
7.1.2.3.4.1.1
7.1.2.3.4.2 Example
7.1.2.3.4.2.1
7.1.2.3.5 Tuberosity
7.1.2.3.5.1 Description
7.1.2.3.5.1.1 A
large, rounded, usually roughened process
7.1.2.3.5.2 Example
7.1.2.3.5.2.1 Ischial
tuberosity of the coxal (hip) bone
7.1.2.3.6
Trochanter
(trō-KAN-ter)
7.1.2.3.6.1 Description
7.1.2.3.6.1.1
7.1.2.3.6.2 Example
7.1.2.3.6.2.1
7.1.2.3.7
Line
7.1.2.3.7.1 Description
7.1.2.3.7.1.1
7.1.2.3.7.2 Example
7.1.2.3.7.2.1
7.1.2.3.8
Spinous
Process (spine)
7.1.2.3.8.1 Description
7.1.2.3.8.1.1
7.1.2.3.8.2 Example
7.1.2.3.8.2.1
7.1.2.3.9
Epicondyle
(epi=above)
7.1.2.3.9.1 Description
7.1.2.3.9.1.1
7.1.2.3.9.2 Example
7.1.2.3.9.2.1
7.1.3
Divisions
of the Skeletal System
7.1.3.1
Skull
7.1.3.1.1
Sutures
7.1.3.1.2
Fontanels
7.1.3.1.3
Cranial Bones
7.1.3.1.3.1 Frontal Bone
7.1.3.1.3.2 Parietal Bones
7.1.3.1.3.3 Temporal Bones
7.1.3.1.3.4 Occipital Bone
7.1.3.1.3.5 Sphenoid Bone
7.1.3.1.3.6 Ethmoid Bone
7.1.3.1.3.7 Facial Bones
7.1.3.1.3.8 Nasal Bones
7.1.3.1.3.9 Maxillae
7.1.3.1.3.10
Paranasal Sinuses
7.1.3.1.3.11
Zygomatic Bones
7.1.3.1.3.12
Mandible
7.1.3.1.3.13
Lacrimal Bones
7.1.3.1.3.14
Palatine Bones
7.1.3.1.3.15
Inferior Nasal Conchae
7.1.3.1.3.16
Vomer
7.1.3.1.4
Orbits
7.1.3.1.5
Foramina
7.1.3.2
Hyoid
Bone
7.1.3.3
Vertebral
Column
7.1.3.3.1
Divisions
7.1.3.3.2
Normal Curves
7.1.3.3.3
Typical
Vertebra
7.1.3.3.4
Cervical
Region
7.1.3.3.5
Thoracic
Region
7.1.3.3.6
Lumbar Region
7.1.3.3.6.1.1
The
lumbar vertebrae, five in number, are large and massive because of their
weight-bearing function and are chiefly characterized by having no foramen in
their transverse processes and no articular facets on their vertebral bodies.
The lumbar curve is convex anteriorly with interposing fibrocartilaginous discs
for mobility.
7.1.3.3.6.1.2
The
spinal foramen in the lumbar region is triangular though smaller than in the
cervical region. The body of each lumbar vertebra is narrower from before
backward and wider from side to side. It is slightly taller anteriorly than
posteriorly, and shows more concavity above than below. The pedicles arise from
either side of the upper portion of the vertebral body. They are short, strong
and proceed directly backward with grooves above and below forming the superior
and inferior vertebral notches. These join to form foraminae for the exit of
the spinal nerves.
7.1.3.3.6.1.3 Springing from the pedicles, are the
short, broad and powerful laminae, which meet in the midline to form the
spinous process. This process is thick and. broad and directed backward in an
almost horizontal direction.
7.1.3.3.6.1.4
At the
base of the laminae are the articular facets. The superior articular facet is
concave and directed medially and backward whereas the inferior articular facet
is directed forward and laterally. The transverse processes, which arise from
the pedicles, are comparatively slender and are situated in front of the
articular 27 processes.
7.1.3.3.6.1.5
On the
posterior superior aspect of the articular facets are slight elevations called
mammillary processes. A similar elevation on the posterior aspect of the
transverse process is called the accessory process.
7.1.3.3.6.1.6
The
intervertebral disc, a fibrocartilaginous structure, acts as a cushion between
the adjacent vertebral bodies. It varies in size and shape and conforms to the
corresponding vertebral body. These discs are thickest in the lumbar region,
increasing in size as they approach the sacrum.
7.1.3.3.6.1.7 The fibrocartilaginous tissue is
concentrically arranged with a thick and tough outer layer called the annulus
fibrosus and a soft gelatinous pulpy substance called the nucleus pulposus,
which are the remains of the notochord. The intervertebral discs are
particularly poor in blood supply.
7.1.3.3.7
Sacrum and
Coccyx
7.1.3.3.7.1.1
In the
adult, the sacrum articulates with the fifth lumbar vertebra, the coccyx and
the two hipbones. It is large, triangular and wedge-shaped and forms the
posterior wall of the pelvis. Its base is oval-shaped and articulates with the
inferior aspect of the body of the fifth lumbar vertebra, forming the prominent
sacral vertebral angle. On either side of the sacrum are triangular areas
called the alae. Just below and to either side of the base is a ridge forming
the sacral portion of the pelvic brim. The lower portion of the sacrum, the
apex, articulates with the coccyx.
7.1.3.3.7.1.2
The
pelvic surface of the sacrum is concave in both dimensions, vertically and
horizontally. At its middle, it is grooved with four transverse ridges, which
mark the points of fusion of the original five vertebrae. There are four
anterior sacral foraminae on either side of the ridges, which permit passage of
the anterior division of the sacral nerve and the lateral sacral arteries.
7.1.3.3.7.1.3 A cross-sagittal section of the
sacrum demonstrates the sacral canal which en- closes the lower portion of the
dura and contents, the roots of the cauda equina, the filum terminale externum,
fat, areolar tissue, blood vessels and fine nerve filaments.
7.1.3.3.8
Thorax
7.1.3.3.8.1 Sternum
7.1.3.3.8.2 Ribs
8.1
Pectoral
(Shoulder) Girdle
8.2
Upper
Extremity
8.3
Pelvic
(Hip) Girdle
8.4
Lower
Extremity
8.4.1
8.4.2
Femur
8.4.3
Patella
8.4.4
Tibia
and Fibula
8.4.4.1
Tibia
8.4.4.1.1 The tibia, or shinbone, is the larger, medial
bone of the leg (Figure 8-12). It bears the major portion of the weight of the
leg. The tibia articulates at its proximal end with the femur and fibula, and
at its distal end with the fibula of the leg and talus bone of the ankle.
8.4.4.1.2 Figure
Photograph of right tibia and fibula (a) Anterior View (b) Posterior View
8.4.4.1.2.1 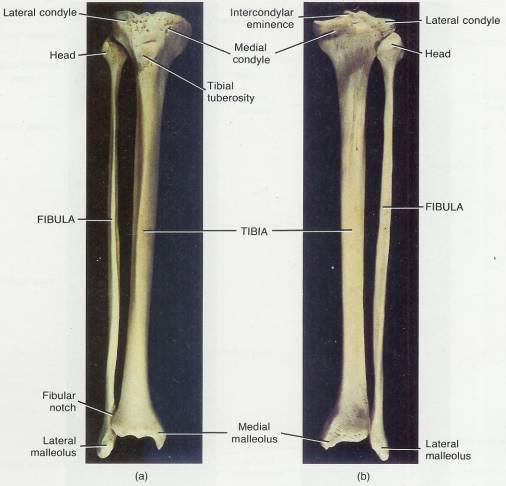
8.4.4.1.3 The
proximal end of the tibia is expanded into a lateral condyle and a medial
condyle. These articulate with the condyles of the femur. The inferior
surface of the lateral condyle articulates with the head of the fibula. The
slightly concave condyles are separated by an upward projection called the intercondylar eminence. The tibial tuberosity on the anterior
surface is a point of attachment for the patellar ligament.
8.4.4.1.4 The
medial surface of the distal end of the tibia forms the medial malleolus (mal-LĒ-ō-lus).
This structure articulates with the talus bone of the ankle and forms the
prominence that can be felt on the medial surface of your ankle. The fibular notch articulates with
the fibula.
8.4.4.2
Fibula
8.4.4.2.1
The fibula is parallel and lateral
to the tibia. It is considerably smaller than the tibia and is non-weight
bearing. The head of
the fibula, the proximal end, articulates with the inferior surface of the
lateral condyle of the tibia below the level of the knee joint. The distal end
has a projection called the lateral
malleolus that articulates with the talus bone of the ankle. This forms
the prominence on the lateral surface of the ankle. The inferior portion of the
fibula also articulates with the tibia at the fibular notch. A fracture of the
lower end of the fibula with injury to the tibial articulation is called a Pott's fracture.
8.4.5
Tarsals,
Metatarsals, and Phalanges
8.4.5.1
Tarsus
(Tarsals) (7) (tahr’sus)
8.4.5.1.1
The tarsus is
a collective designation for the seven bones of the ankle called tarsals
(Figure 8-13). The term tarsos pertains to a broad, flat surface.
8.4.5.1.2
Figure 8-13
Photograph of the right foot in superior view
8.4.5.1.2.1
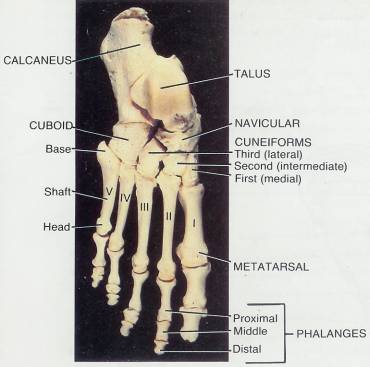
8.4.5.1.3 The talus and calcaneus (kal-KĀ-nē-us)
are located on the posterior part of the foot. The anterior part contains the cuboid, navicular, and three cuneiform (cuneiform
= wedge-shaped) bones
called the first (medial), second (intermediate), and third (lateral) cuneiform. The
talus, the uppermost tarsal bone, is the only bone of the foot that articulates
with the fibula and tibia. It is surrounded on one side by the medial malleolus
of the tibia and on the other side by the lateral malleolus of the fibula.
During walking, the talus initially bears the entire weight of the body. About
half the weight is then transmitted to the calcaneus. The remainder is
transmitted to the other tarsal bones. The calcaneus, or heel bone, is the
largest and strongest tarsal bone.
8.4.5.2
Metatarsals
8.4.5.2.1 The metatarsus consists of five
metatarsal bones numbered I to V from the medial to lateral position. Like the
metacarpals of the palm of the hand, each metatarsal consists of a proximal base, a shaft, with a distal head. The metatarsals articulate
proximally with the first, second, and third cuneiform bones and with the
cuboid. Distally, they articulate with the proximal row of phalanges. The first
metatarsal is thicker than the others because it bears more weight.
8.4.5.3
Phalanges
8.4.5.3.1
The phalanges
of the foot resemble those of the hand both in number and arrangement.
Each also consists of a proximal base,
a middle shaft,
and a distal head.
The hallux (great or big
toe), has two large, heavy phalanges called proximal and distal phalanges. The
other four toes each have three phalanges-proximal, middle, and distal.
8.4.6
Arches
of the Foot
8.4.6.1
The
bones of the foot are arranged in two arches (Figure 8-14). These arches enable
the foot to support the weight of the body, provide an ideal distribution of body
weight over the hard and soft tissues of the foot, and provide leverage while
walking.
8.4.6.2
Figure
8-14 Arches of the right foot in lateral View
8.4.6.2.1
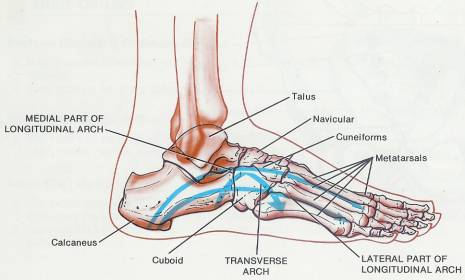
8.4.6.3
The
arches are not rigid. They yield as
weight is applied and spring back when the weight is lifted, thus helping to
absorb shocks.
8.4.6.4
The
longitudinal arch has two parts. Both consist of tarsal and metatarsal bones
arranged to form an arch from the anterior to the posterior part of the foot.
The medial (inner) part of the longitudinal arch originates at the calcaneus.
It rises to the talus and descends through the navicular, the three cuneiforms,
and the heads of the three medial metatarsals. The talus is the keystone of
this arch. The lateral (outer) part of the longitudinal arch also begins at the
calcaneus. It rises at the cuboid and descends to the heads of the two lateral
metatarsals. The cuboid is the keystone of this arch.
8.4.6.5
The
transverse arch is formed by the navicular, three cuneiforms, cuboid, and the
bases of the five metatarsals.
8.4.7
Clinical
Application: Flatfoot, Clawfoot, and Bunions
8.4.7.1
The
bones composing the arches are held in position by ligaments and tendons. If
these ligaments and tendons are weakened, the height of the medial longitudinal
arch may decrease or “fall”. The result is flat foot.
8.4.7.2
Clawfoot
is a condition in which the medial longitudinal arch is abnormally elevated. It
is frequently caused by muscle imbalance, such as may result from
poliomyelitis.
8.4.7.3
A
bunion (hallux valgus; valgus bent outward) is a deformity of the great toe.
Although the condition may be inherited, it is typically caused by wearing
tightly fitting shoes and is characterized by lateral deviation of the great
toe and medial displacement of metatarsal 1. Arthritis of the first
metatarsophalangeal joint may also be a predisposing factor. The condition
produces inflammation of the bursae (fluid-filled sacs at the joints), bone
spurs, and calluses.
8.5
Female
and Male Skeletons
9.1 Introduction
9.1.1 Bones are too rigid to bend without causing damage. Fortunately, the
skeletal system consists of many separate bones, most of which are held
together at joints by flexible connective tissue. All movements that change the
positions of the bone parts of the body occur at joints. You can understand the
importance of joints if you imagine how a cast over the knee joint prevents
flexing the leg or how a splint on a finger limits the ability to manipulate
small objects.
9.1.2 An articulation (joint) is a point of contact between bones between
cartilage and bones, or between teeth and bones. The scientific study of joints
is referred to as Arthrology (ar-THROL-ō-jē; arthro=joint;
logos=sturdy of). The joint’s structure determines how it functions. Some
joints permit no movement, other permit slight movement, and still others
afford considerable movement. In general, the closer the fit at the point of
contact, the stronger the joint. At tightly fitted joints, however, movement is
restricted. The looser the fit, the greater the movement. Unfortunately,
loosely fitted joints are prone to dislocation. Movement at joints is also
determined by the structure (shape) of the articulation bones, the flexibility
(tension or tautness) of the connective tissue ligaments and joint capsules
that bind the bones together, and the position of ligaments, muscles, and
tendons.
9.2 Classification of Joints
9.2.1 Functional
9.2.1.1
Introduction
9.2.1.1.1 The functional classification of joints
takes into account the degree of movement they permit.
9.2.1.2
Synarthroses
(sin-ar-THRŌ-sēz))
9.2.1.2.1 Immovable
9.2.1.3
Amphiarthroses (am-fē
–ar-THRŌ-sēz)
9.2.1.3.1 Slightly moveable
9.2.1.4
Diarthroses (dī-ar-THRŌ-sēz)
9.2.1.4.1 Freely movable
9.2.2 Structural
9.2.2.1
The structural classification of
joints is based on the presence or absence of a synovial (joint) cavity (a
space between the articulating bones) and the kind of connective tissue that
binds the bones together. Structurally, joints are classified as Fibrous Joints, in which there is no
synovial cavity and the bones are held together by fibrous connective tissue. Cartilaginous Joints have no
synovial cavity and the bones are held together by cartilage. Synovial Joints have a synovial
cavity and the bones forming the joint are united by a surrounding articular
capsule and frequently by accessory ligaments (described later). We will
discuss the joints of the body based upon their structural classification, but
with reference to their functional classification as well.
9.3 Fibrous Joints
9.3.1 Fibrous joints lack a synovial cavity, and the articulating bones
are held very closely together by fibrous connective tissue. They permit little
or no movement. The three types of fibrous joints are Sutures, Syndesmoses, and
gomphoses.
9.3.2 Suture
9.3.2.1
9.3.3 Syndesmosis (Sin’-dez-MŌ-sis)
9.3.3.1
This is a fibrous joint in which the
uniting fibrous connective tissue is present in a much greater amount than in a
suture, but the fit between the bones is not quite as tight.
9.3.3.2
The fibrous connective forms an
interosseous membrane or ligament.
9.3.3.3
A Syndesmosis is slightly movable
because the bones are separated more than in a suture and some flexibility is
permitted by the interosseous membrane or ligament.
9.3.3.4
Syndesmoses are functionally
classified as amphiarthrotic and typically permit slight movement.
9.3.3.5
An example of a Syndesmosis is the
distal articulation of the fibia and fibula
9.3.4 Gomphosis
9.3.4.1
9.4 Cartilaginous Joints
9.4.1
9.4.2 Synchondrosis
9.4.2.1
9.4.3 Symphysis
9.4.3.1
9.5 Synovial Joints (si-NŌ-vē-al)
9.5.1 Structure
9.5.1.1
A
joint in which there is a space between articulating bones is called a synovial
joint. The space is called a synovial (joint) cavity. Because of this cavity
and because of the arrangement of the articular capsule and accessory
ligaments, synovial joints are freely movable. Thus, synovial joints are
functionally classified as diarthrotic.
9.5.1.2
Synovial
joints are different from the other joint types in the following ways.
9.5.1.2.1 Synovial
Joint Cavity
9.5.1.2.1.1 When a joint has a
space between its cartilaginous end surfaces, it is called a Synovial
(si-NŌ-vē-al) joint.
9.5.1.2.1.2 This space is
called a Synovial (joint) cavity. This cavity along with the articular capsule
and its accessory ligaments allows Synovial joints to be freely movable.
9.5.1.2.2 Hyaline
(Articular) Cartilage
9.5.1.2.2.1 The hyaline
(articular) cartilage covering the end surfaces of bones is another distinctive
feature of Synovial joints. It provides a smooth, relatively friction free
surface around which bones can move unimpeded.
9.5.1.2.3 Articular
Capsule
Back Table
of Contents References
9.5.1.2.3.1 The articulating
bones are united by this sleeve like capsule, which is composed of two layers
as follows.
9.5.1.2.3.1.1 Fibrous Capsule
9.5.1.2.3.1.1.1
The fibrous capsule is the outermost layer of the articular
capsule.
9.5.1.2.3.1.1.2
The fibrous capsule is composed of dense connective
(collagenous) tissue.
9.5.1.2.3.1.1.3
It is attached to the periosteum of articulating bones at
variable distance from the edge of the articular cartilage.
9.5.1.2.3.1.1.4
It is flexible so that it permits movement at the joint. It
has great tensile strength so that it resists dislocation.
9.5.1.2.3.1.1.5
Some fibrous capsules have outer layers of its fibers arranged
in parallel bundles called ligaments, which are individually named.
9.5.1.2.3.1.1.6
Ligaments resist repetitive strain and bind bone to bone.
9.5.1.2.3.1.2 Synovial
Membrane
9.5.1.2.3.1.2.1
This is the innermost layer of the articular capsule and is
another distinguishing feature of Synovial joints.
9.5.1.2.3.1.2.2
It is composed of a variable amount of adipose (fat) and loose
connective tissue with elastic fibers.
9.5.1.2.3.1.2.3
The Synovial membrane secretes Synovial fluid (SF), which
provides both nourishment to the articular cartilage and lubrication to the
joint.
9.5.1.2.3.1.2.4
Phagocytic cells found
in the Synovial fluid remove microbes and dead cells (debris) caused by wear
and tear on the joint.
9.5.1.2.3.1.2.5
The Synovial fluid consists of hyaluronic acid and an
interstitial fluid produced from blood plasma.
9.5.1.2.3.1.2.6
Synovial fluid is similar in consistency to uncooked egg
white, which is viscous when there is no joint movement and less viscous with
increased joint movement.
9.5.1.2.3.1.2.7
There is just enough Synovial fluid in the joint to form a
thin viscous layer over the joint surfaces. The thin film reduces friction and
supplies nutrients and removes metabolic waste from the cartilage cells of the
articulate cartilage. The amount of synovial fluid will vary from joint to
joint. The amount of Synovial fluid in a large joint such as the knee is about
3.5 ml (0.1 oz).
9.5.1.3
Crackling Sound
Back Table
of Contents References
9.5.1.3.1 When
some synovial joints are pulled apart, they make a crackling sound. As space is created in a joint, negative
pressure develops in the synovial fluid driving out carbon dioxide. As a result a gas bubble develops. As the opposing articular surfaces are
pulled apart further and then abruptly, separate the pressure within the joint
exceeds that in the bubble and the bubble collapses, producing a crackling
noise. The collapse of the large bubble
creates a series of smaller bubbles, which gradually go back into
solution. Until the small bubbles
disappear and the gas is completely dissolved, the joint cannot be cracked
again. Increased congruence of joint
surfaces such as those found between the phalanges and metacarpals, crack more
easily than the joint surfaces that are less congruent.
9.5.1.4
Accessory ligaments
9.5.1.4.1 There
are two kinds of accessory ligaments (ligare= to bind) one which is on the
outside of the joint capsule is named the extracapsular ligament and another is
named the intracapsular ligament because it is on the inside of a joint
capsule.
9.5.1.4.2 Extracapsular
Ligaments
9.5.1.4.2.1 These accessory
ligaments are outside the articular capsule.
An example would be the fibular collateral ligament of knee joint.
9.5.1.4.3 Intracapsular
Ligaments
9.5.1.4.3.1 These accessory
ligaments are within the articular capsule but excluded from the synovial
cavity by reflections of a synovial membrane.
The cruciate ligaments within the knee joint are an example.
9.5.1.5
Articular Discs (Menisci)
9.5.1.5.1 The
menisci are pads of fibrocartilage between the articular surfaces of the
bone. They attach to the margins of the
fibrous capsule. The menisci subdivide the
space between the joint into two separate areas and allow two bones of
different shapes to fit snugly. This
helps maintain the stability of the joint while also directing the synovial
fluid to areas of greatest friction.
9.5.1.6
Bursae
Back Table
of Contents References
9.5.1.6.1 Bursae
help reduce friction between moving parts.
Bursae are saclike structures whose walls consist of connective tissue
similar to the fibrous tissue around the joint capsule. Bursae are lined with a
synovial membrane and filled with fluid similar to synovial fluid. Bursae cushion movement of one part of a
body over another and repetitive stress can inflame them. This inflammation is called bursitis. Bursae are found between the following
structures.
9.5.1.6.1.1 Skin and bone in
places where the skin rubs over the bone.
9.5.1.6.1.2 Tendons and bones.
9.5.1.6.1.3 Muscles and bones
9.5.1.6.1.4 Ligaments and
bones
9.5.1.7
Joint Stability
9.5.1.7.1 Joint
stability requires that bones remain in contact with each other. Bone to bone contact is facilitated by
several factors as follows;
9.5.1.7.2 Fit of
articulating bones
9.5.1.7.2.1 In the hip joint,
for example, the head of the femur and the acetabulum of the coxal (hip) bone
interlock with one another.
9.5.1.7.3 Strength
and tension (tautness) of the joint ligaments
9.5.1.7.3.1 The thick
ligaments of the coxal (hip) joint help provide stability to the articulating
bones.
9.5.1.7.4 Tension
and arrangement of muscles around the joint
9.5.1.7.4.1 The knee joint,
for example, is surrounded by a fibrous capsule which is formed primarily from
the tendinous expansions of the muscles acting on the joint.
9.5.1.8
Medical Tests, Procedures, medical
conditions and definitions
Back Table
of Contents References
9.5.1.8.1 Arthrocentesis
(ar-thrō-sen-TĒ-sis; arthro= joint; centesis= to puncture) (joint
tap)
9.5.1.8.1.1 Diagnostic and or
Therapeutic value
9.5.1.8.1.1.1 This
procedure removes a sample of synovial fluid for analysis. The sample once analyzed helps diagnose the
cause of joint swelling, inflammation, and or pain.
9.5.1.8.1.1.2 Fluid
is drained from the joint capsule. To
decrease the pressure within the joint capsule caused by a build up of synovial
fluid, blood, and/or pus.
9.5.1.8.1.1.3 Local
anesthesia or steroid medication is injected into the joint capsule. This procedure helps reduce the pain of
arthritis.
9.5.1.8.1.1.4 Abnormal
joint fluid is drained from the capsule and the joint is cleansed by saline
irrigations.
9.5.1.8.1.2 Procedure
9.5.1.8.1.2.1 Antiseptic
solution is applied to the skin over the joint for cleansing.
9.5.1.8.1.2.2 An
aesthetic is injected into the area.
9.5.1.8.1.2.3 A
sample of synovial fluid is obtained by inserting a needle into the synovial
joint cavity.
9.5.1.8.1.2.4 Fluid
analysis is completed for cell content, which includes urate and other
crystals.
9.5.1.8.1.2.5 Color
and viscosity are also noted.
9.5.1.8.1.2.6 A
sample is submitted for microbial culture and chemical analysis, which includes
glucose, protein, and enzymes.
9.5.1.8.2 Artificial
Ligaments and Tendons
Back Table
of Contents References
9.5.1.8.2.1 These ligaments
and tendons are used to replace ligaments and tendons, which are damaged. An example of this would be the carbon fiber
implant. Carbon fibers are coated with a
plastic called polylactic acid.
Ligaments and tendons are then reinforced by these coated fibers, which
are sewn in and around them. The
polylactic acid is absorbed within about two weeks by the body and the carbon
fibers eventually fracture. As the artificial fibers either are absorbed by the
body or break apart, they are surrounded by collagen produced by fibroblasts.
9.5.1.8.3 Torn
cartilage
9.5.1.8.3.1 Articular disks
(menisci) are torn (torn cartilage) usually during athletic activity. Surgical removal (meniscectomy) is necessary
to avoid wear and tear, which may result in arthritis. This surgery, which once involved removal of
much if not all of the cartilage, also damaged healthy tissue and required
extensive time-consuming rehabilitation.
Arthroscopy reduces the damage to tissue and shortens rehabilitation
time.
9.5.1.8.4 Arthroscopy
(ar-THROS-kō-pē; arthro= joint; skopein= to view)
9.5.1.8.4.1 An arthroscope is
a lighted instrument about the diameter of the pencil, which is used to examine
the interior of the joint usually a knee.
9.5.1.8.4.2 Diagnostic value
9.5.1.8.4.2.1 Arthroscopy
provides internal Assessment of the damage following a knee injury.
9.5.1.8.4.2.2 Arthroscopy
helps remove torn cartilage, and repair cruciate ligaments within the joint
capsule.
9.5.1.8.4.2.3 Tissue
samples can be obtained and analyzed.
9.5.1.8.4.2.4 Disease
progression and set the effects can be monitored.
9.5.1.8.4.2.5 Surgical
procedures can be planned.
9.5.1.8.4.3 Procedure
9.5.1.8.4.3.1 A
local or general anesthetic is administered.
9.5.1.8.4.3.2 A
quarter inch incision is made and the arthroscope is inserted.
9.5.1.8.4.3.3 A
tube for the saline solution is inserted in another small incision. The arthroscope itself can also introduce saline
solution.
9.5.1.8.4.3.4 An
additional small incision allows for the insertion of an instrument that shaves
off, reshapes the damaged cartilage, and removes the shaved cartilage along
with the salt solution.
9.5.1.8.4.3.5 A
video camera can be attached to the arthroscope for real time screen projection
or later viewing.
9.5.1.8.4.3.6 Because
of the small incision is recovery time is much improved over methods that are
more conventional.
9.5.1.8.4.3.7 The
patient may wear a cast or split for several days depending on the extent of
the procedure
9.5.2 Movements
9.5.2.1
The movements permitted at synovial
joints are limited by several factors.
9.5.2.2
Structure (Shape) of the Articulating
Bones
9.5.2.2.1 The
shape of the articulating bones and how they fit together will determine the
movements permitted at that synovial joint.
9.5.2.3
Tension of Ligaments
9.5.2.3.1 The
different components of a fibrous capsule are tense only when the joint is in
certain positions. Tense ligaments not only restrict the range of movement but
also direst the movement of the articulating bones with respect to each other.
In the knee joint, for example, the major ligaments are lax when the knee is
bent but tense when the knee is straightened. Also, when the knee is
straightened, the surfaces of the articulating bones are in fullest contact
with each other.
9.5.2.4
Muscle Arrangement and Tension
9.5.2.4.1 How
the muscles are arranged and the tension they place on the join, which
reinforce the restraint placed on a joint by ligaments is another factor which
restricts movement at a synovial joint.
9.5.2.5
Apposition of Soft Parts
9.5.2.5.1 This
may limit mobility. For example, during bending at the elbow, the anterior
surface of the forearm is pressed against the anterior surface of the arm.
9.5.2.6
Gliding
9.5.2.6.1 A
gliding movement is the simplest kind that can occur at a joint. One surface
moves back and forth and from side to side over another surface without angular
or rotary motion. Some joints that glide are those between the carpals and
between the tarsals. The heads and tubercles of the ribs glide on the bodies
and transverse processes of the vertebrae.
9.5.2.7
Angular
9.5.2.7.1 Increase
or decrease the angle between bones. Among the angular movements are flexion,
extension, abduction, and adduction.
9.5.2.7.2 Flexion
9.5.2.7.2.1 Decreases the
angle between the surfaces of the articulating bones. Examples of flexion
include bending the head forward (the joint is between the occipital bone and
the atlas), bending the elbow, and bending the knee.
9.5.2.7.3 Extension
9.5.2.7.3.1 Involves an
increase in the angle between the surfaces of the articulating bones. Extension
restores a body part to its anatomical position after it has been flexed. Examples
of extension are returning the head to the anatomical position after flexion,
straightening the arm after flexion, and straightening the leg after flexion.
Continuation of extension beyond the anatomical position, as in bending the
head backward, is called hyperextension.
9.5.2.7.4 Abduction
9.5.2.7.4.1 Movement of a bone
away from the midline of the body. An example of abduction is moving the arm
upward and away from the body until it is held straight out at right angles to
the chest. With the fingers and toes, however, the midline of the body is not
used as the line of reference. Abduction of abduction of the fingers (not the
thumb) is a movement away from an imaginary oline drawn through the middle
finger; in other words, is is spreading the fingers. Abduction of the thumb
moves the thumb away from the plane of the palm at a right angle to the palm.
Abduction of the toes is relative to an imaginary line drawn through the second
toe.
9.5.2.7.4.2 Further Reading
9.5.2.7.4.2.1
Adduction and Abduction
9.5.2.7.5 Adduction
9.5.2.7.5.1 Movement of a part
toward the midline of the body. An example of adduction is returning the arm to
the side after abduction. As in abduction, adduction of the fingers (not the
thumb) is relative to the middle finger, and adduction of the toes is relative
to the second toe. In adduction of the thumb, the thumb moves toward the plane
of the palm at a right angle to the palm.
9.5.2.7.5.2 Further Reading
9.5.2.7.5.2.1
Adduction and Abduction
9.5.2.8
Rotation
9.5.2.8.1 Movement
of a bone around its own longitudinal axis. During rotation, no other motion is
permitted.
9.5.2.8.1.1 Medial Rotation
9.5.2.8.1.1.1 Anterior
surface of a bone or extremity moves toward the midline.
9.5.2.8.1.2 Lateral Rotation
9.5.2.8.1.2.1 Anterior
surface moves away from the midline.
9.5.2.8.2 We
rotate the atlas around the dens of the axis when we shake the head from side
to side. Rotation of the humerus turns the anterior surface of the humerus to
face wither medially or laterally.
9.5.2.9
Circumduction
9.5.2.9.1 Movement
in which the distal end of a bone moves in a circle while the proximal end
remains stable. The bone describes a cone in the air. Circumduction typically
involves flexion, abduction, adduction, extension, and rotation. It involves a
360° rotation. An example is moving the outstretched arm in a circle toward up
to pitch a ball.
9.5.2.10
Special
9.5.2.10.1
Special movements are those found only at the joints indicated
9.5.2.10.1.1
Inversion
9.5.2.10.1.1.1
Movement of the sole
of the foot inward (medially) so that the soles face toward each other.
9.5.2.10.1.1.2
Further Reading
9.5.2.10.1.1.2.1
Inversion
9.5.2.10.1.2
Eversion
9.5.2.10.1.2.1
Movement of the sole outward (laterally) so that the soles
face away from each other
9.5.2.10.1.2.2
Further Reading
9.5.2.10.1.2.2.1
Eversion
9.5.2.10.1.3
Dorsiflexion
9.5.2.10.1.3.1
Involves bending of the foot in the direction of the dorsum
(upper surface) of the foot.
9.5.2.10.1.4
Plantar Flexion
9.5.2.10.1.4.1
Involves bending the foot in the direction of the plantar
surface (sole) of the foot.
9.5.2.10.1.4.2
Further Reading
9.5.2.10.1.4.2.1
Flexion and Extension
9.5.2.10.1.5
Protraction
9.5.2.10.1.5.1
Movement of the mandible or shoulder girdle forward on a plane
parallel to the ground. Thrusting the jaw outward is protraction of the
mandible. Bring your arms forward until the elbows touch requires protraction
of the clavicle or shoulder girdle.
9.5.2.10.1.6
Retraction
9.5.2.10.1.6.1
Movement of a protracted part of the body backward on a plane
parallel to the ground. Pulling the lower jaw back in line with the upper jaw
is retraction of the mandible.
9.5.2.10.1.7
Supination
9.5.2.10.1.7.1
Movement of the forearm in which the palm of the hand is
turned anterior or superior. To demonstrate supination, flex your forearm at
the elbow to prevent rotation of the humerus in the shoulder joint.
9.5.2.10.1.7.2
Further Reading
9.5.2.10.1.7.2.1
Supination and Pronation
9.5.2.10.1.7.2.2
9.5.2.10.1.8
Pronation
9.5.2.10.1.8.1
Movement of the forearm in which the palm is turned posterior
or ingerior.
9.5.2.10.1.8.2
Further Reading
9.5.2.10.1.8.2.1
Supination and Pronation
9.5.2.10.1.9
Elevation
9.5.2.10.1.9.1
Upward movement of a part of the body. You elevate your
mandible when you close your mouth.
9.5.2.10.1.10
Depression
9.5.2.10.1.10.1
Downward movement of a part of the body. You depress your
mandible when you open your mouth. The shoulders can also be elevated and
depressed.
9.5.3 Types of Synovial Joints
Back Table
of Contents References
9.5.3.1
Types
9.5.3.1.1 The
shape of the articulating bones determines the type of movement that can be
performed. The structure of synovial
joints although similar differ in articular shape and can be divided into six
sub-types as follows; Gliding, Hinge, Pivot, Ellipsoidal, Saddle, and Ball and
Socket.
9.5.3.2
Gliding
9.5.3.2.1 The
bones of gliding joints (arthrodia (ar-THRŌ-dē-a)) form a flat
surface so that only side-to-side and back and fourth movements are
possible. The ligaments or adjacent
bones limit twisting and rotation movements.
Gliding joints are nonaxial because there's no axis around which they
move.
9.5.3.2.2 Movements
9.5.3.2.2.1 Gliding
9.5.3.2.3 Examples
9.5.3.2.3.1 Joints between the
carpal bones
9.5.3.2.3.2 Tarsal bones
9.5.3.2.3.3 Sternum and
clavicle
9.5.3.2.3.4 Scapula and
clavicle
9.5.3.3
Hinge
9.5.3.3.1 The
hinge or ginglymus (JIN-gli-mus) joint is one in which the concave surface
of one bone fits into the convex surface of another bone. This joint is monaxial or uniaxial because
its movement is primarily a single plane.
A door hinge is a good example of this type of joint.
9.5.3.3.2 Movements
9.5.3.3.2.1 Flexion
9.5.3.3.2.2 Extension
9.5.3.3.3 Examples
9.5.3.3.3.1 Elbow
9.5.3.3.3.2 Ankle
9.5.3.3.3.3 Interphalangeal
joints
9.5.3.4
Pivot
Back Table
of Contents References
9.5.3.4.1 A
pivot or trochoid (TRŌ-koyd) is formed between the rounded, pointed,
or conical surface of one bone, which articulates within a ring, formed partly
by another bone and partly by ligament.
This joint is monaxial in that the primary movement permitted is
rotation.
9.5.3.4.2 Movements
9.5.3.4.2.1 Supination and
pronation of the palms
9.5.3.4.2.2 Rotation of the
head from side to side
9.5.3.4.3 Examples
9.5.3.4.3.1 Atlas and axis
(atlantoaxial)
9.5.3.4.3.2 Proximal ends of
the Radius and ulna
9.5.3.5
Ellipsoidal
9.5.3.5.1 An ellipsoidal
or condyloid (KON-di-loyd) joint, an oval-shaped condyle of one bone fits
into an elliptical cavity of another bone. Since the joint permits side-to-side
and back-and-forth movements, it is biaxial. The joint at the wrist between the
radius and carpals is ellipsoidal. The movement permitted by such a joint is
illustrated when you flex and extend and abduct and adduct and circumduct the
wrist.
9.5.3.5.2 Movements
9.5.3.5.2.1 Flex and extend
and abduct and adduct and circumduct the wrist.
9.5.3.5.3 Examples
9.5.3.5.3.1 Wrist
9.5.3.5.3.2 Metatarsophalangeal
Joints (MP)
9.5.3.6
Saddle (Reciprocal Reception)
9.5.3.6.1 In a saddle
or sellaris (sel-A-ris) joint, the articular surface of one bone is
saddle-shapped and the articular surface of the other bone is shaped like a
rider sitting in the saddle. Essentially, the saddle joint is a modified
ellipsoidal joint in which the movement is somewhat freer. Movements at a
saddle joint are die to side and back and forth. Thus, the joint is biaxial.
9.5.3.6.2 Movements
9.5.3.6.2.1 Side to side and
back and forth
9.5.3.6.3 Examples
9.5.3.6.3.1 Trapezium of the
carpus and the metacarpal of the thumb
9.5.3.6.3.2 Calcaneocuboid
Joint of foot
9.5.3.7
Ball and Socket
9.5.3.7.1 A ball
and socket or spheroid (SFĒ-royd) joint consists of a ball-like
surface of one bone fitted into a cuplike depression of another bone. Such a
joint permits triaxial movement, or movement in three planes of motion:
flexion-extension, abduction-adduction, and rotation-Circumduction. The range
of movement at a ball-and-socket joint is illustrated by Circumduction of the
arm.
9.5.3.7.2 Movements
9.5.3.7.2.1 Flexion-extension,
9.5.3.7.2.2 Abduction-adduction
9.5.3.7.2.3 Rotation-Circumduction
9.5.3.7.3 Examples
9.5.3.7.3.1 Shoulder Joint
9.5.3.7.3.2 Coxal (hip) Joint
9.5.3.7.3.3 Talocalcaneonavicular
(TCN) of the foot
9.5.4 Discussion of Selected Synovial Joints
Back Table
of Contents References
9.5.4.1
Humeroscapular (Shoulder) Joint
9.5.4.1.1
9.5.4.1.2 Articular
Capsule
9.5.4.1.3 Coracohumeral
Ligaments
9.5.4.1.4 Transverse
Humeral Ligament
9.5.4.1.5 Transverse
Humeral Ligament
9.5.4.1.6 Glenoid
Labrum
9.5.4.1.7 Bursae
9.5.4.1.7.1 Subscapular Bursa
9.5.4.1.7.1.1
9.5.4.1.7.2 Subdeltoid Bursa
9.5.4.1.7.2.1
9.5.4.1.7.3 Subacromial Bursa
9.5.4.1.7.3.1
9.5.4.1.7.4 Subcoracoid Bursa
9.5.4.1.7.4.1
9.5.4.2
Coxal (Hip) Joint
Back Table
of Contents References
9.5.4.2.1
9.5.4.2.2 Articular
Capsule
9.5.4.2.2.1
9.5.4.2.3 Iliofemoral
Ligament
9.5.4.2.3.1
9.5.4.2.4 Pubofemoral
Ligament
9.5.4.2.4.1
9.5.4.2.5 Ischiofemoral
Ligament
9.5.4.2.5.1
9.5.4.2.6 Capitate
Ligament (Ligament of the head of the femur)
9.5.4.2.6.1
9.5.4.2.7 Acetabular
Labrum
9.5.4.2.7.1
9.5.4.2.8 Transverse
Ligament of the Acetabulum
9.5.4.2.8.1
9.5.4.2.9 Dislocation of the Hip
9.5.4.2.9.1
9.5.4.3
Tibiofemoral (Knee) Joint
Back Table
of Contents References
9.5.4.3.1
9.5.4.3.2 Articular
Capsule
9.5.4.3.2.1
9.5.4.3.3 Medial
and Lateral Patellar Retinacula
9.5.4.3.3.1
9.5.4.3.4 Patellar
Ligament
9.5.4.3.4.1
9.5.4.3.5 Oblique
Popliteal Ligament
9.5.4.3.5.1
9.5.4.3.6 Arcuate
Popliteal Ligament
9.5.4.3.6.1
9.5.4.3.7 Tibial
Collateral Ligament
9.5.4.3.7.1
9.5.4.3.8 Fibular
Collateral Ligament
9.5.4.3.8.1
9.5.4.3.9 Intraarticular
Ligaments
9.5.4.3.9.1 Anterior Cruciate
Ligament
9.5.4.3.9.1.1
9.5.4.3.9.2 Posterior Cruciate
Ligament
9.5.4.3.9.2.1
9.5.4.3.10
Articular Discs
9.5.4.3.10.1
Medial Meniscus
9.5.4.3.10.1.1
9.5.4.3.10.2
Lateral Meniscus
9.5.4.3.10.2.1
9.5.4.3.11
Bursae
Back Table
of Contents References
9.5.4.3.11.1
Anterior Bursae
9.5.4.3.11.1.1
9.5.4.3.11.2
Medial Bursae
9.5.4.3.11.2.1
9.5.4.3.11.3
Lateral Bursae
9.5.4.3.11.3.1
9.5.4.3.12
Knee Injuries
9.5.4.3.12.1
Rupture of Tibial Collateral Ligament, Tearing of Anterior
Cruciate & Medial Meniscus
9.5.4.3.12.1.1
9.5.4.3.12.2
Swollen Knee
9.5.4.3.12.2.1
9.5.4.3.12.3
Dislocated Knee
9.5.4.3.12.3.1
9.5.5 Exhibit 9-3
9.5.5.1
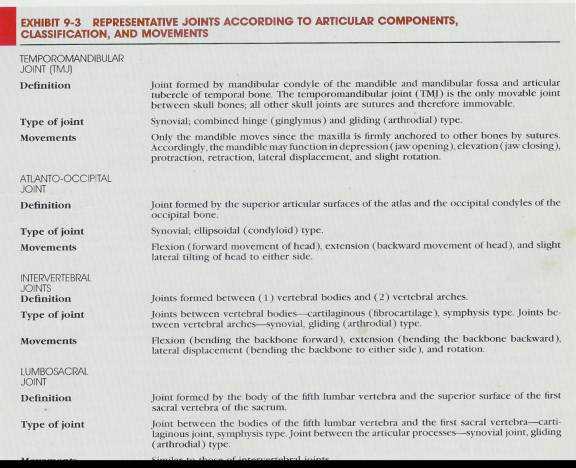
9.5.5.2
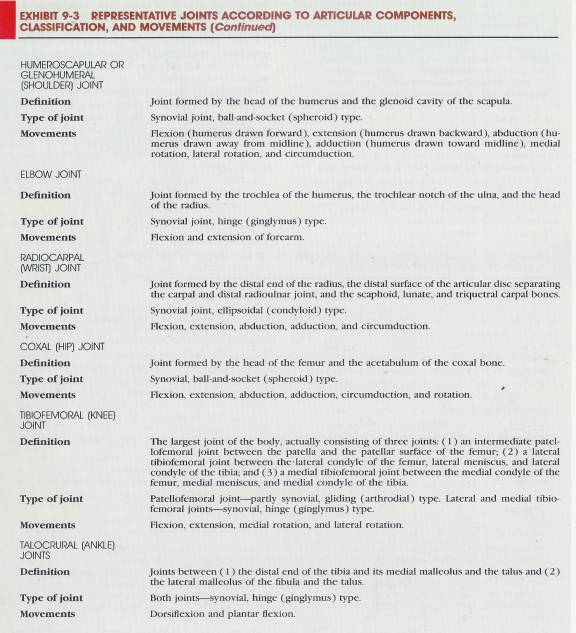
9.6 Disorders: Homeostatic Imbalance
Back Table of
Contents References
9.6.1 Rheumatism (rheumat=Subject to flux)
9.6.1.1
Refers to any painful state of the
supporting structures of the body- its bones, ligaments, joints, tendons, or
muscles. Arthritis is a form of rheumatism in which the joints have become
inflamed.
9.6.2 Arthritis
9.6.2.1
The term arthritis refers to many
different diseases, the most common of which are rheumatoid arthritis (RA),
Osteoarthritis, and gouty arthritis. All are characterized by inflammation of
one or more joints. Inflammation, pain, and stiffness may also be present in
adjacent parts of the body, such as the muscles near the joint.
9.6.3 Rheumatoid Arthritis (RA) (ROO-ma-toyd)
9.6.3.1
This is an autoimmune disease in which
the body attacks its own tissues; in this case its own cartilage and joint
linings. It is characterized by inflammation of the joint, swelling, pain, and
loss of function. Usually, this form occurs bilaterally-if your left wrist is
affected; your right wrist may also be affected, although usually not to the
same degree.
9.6.3.2
Symptom
9.6.3.2.1 The
primary symptom of rheumatoid arthritis is inflammation of the synovial
membrane. If untreated, the following sequential pathology may occur. The
membrane thickens and synovial fluid accumulates. The resulting pressure causes
pain and tenderness. The membrane then produces an abnormal granulation tissue
called a pannus, which adheres to the surface of the articular cartilage. The
pannus formation sometimes erodes the cartilage completely. When the cartilage
is destroyed fibrous tissue joins the exposed bone ends. The tissue ossifies
and fuses the joint so that it is immovable- the ultimate crippling effect of
rheumatoid arthritis. Most cases do not progress to this stage, but the range
of motion of the joint is greatly inhibited by the server inflammation and
w\swelling. The growth of the pannus is shat causes the distortion of the
fingers that is so typical of the clinical appearance of hands that have been
affected by rheumatoid arthritis. A plastic surgeon or orthopedic will remove
the pannus growth before crippling and dysfunctional deformities have
occurred-long before the joints are fused to the point of immobility.
9.6.3.3
Treatment
9.6.3.3.1 Treatment
is aimed at reducing pain and inflammation and preserving muscle strength and
joint function. Therapies include adequate rest; anti-inflammatory drugs, such
as aspirin and in selected patients steroids; exercises to maintain full range
of joint motion; heat and other forms of physical therapy; and weight loss to
relieve pressure on weight-bearing joints. In severe cases, damaged joints may
be surgically replaced, either partly or entirely, with artificial joints. The
artificial parts are inserted after removal of the diseased portion of the
articulating bone and its cartilage. The new metal or plastic joint is fixed in
place with special acrylic cement. When freshly mixed in the operating room, it
hardens as strong as bone in minutes.
9.6.3.4
Osteoarthritis
(os’-tē-ō-ar-THRĪ-tis)
9.6.3.4.1 A
degenerative joint disease far more than rheumatoid arthritis , and usually
less damaging, is Osteoarthritis. It apparently results from a combination of
aging, irritation of the joints and wear and abrasion.
9.6.3.4.2 Degenerative
joint disease is a non-inflammatory, progressive disorder of movable joint,
particularly weight-bearing joints. It is characterized by the deterioration of
articular cartilage and by formation of new bone in the subchondral areas and
at the margins of the joint. The cartilage slowly degenerates, and as the bone
ends become exposed, small bumps, or spurs, of new osseous tissue are deposited
on them. These spurs decrease the space of the joint cavity and restrict joint
movement. Unlike rheumatoid arthritis, Osteoarthritis usually affects only the
articular cartilage. The synovial membrane is rarely destroyed, and other
tissues are unaffected. The main distinction between Osteoarthritis and
rheumatoid arthritis is that the former strides the big joints (knees, hips)
first, whereas the latter strikes the small joints first. But Osteoarthritis
may affect the fingers, and when this is the case, the distal phalanges show
the most prominent changes. The effect of rheumatoid arthritis on the fingers
is most pronounced proximally, in the wrists and in the Metacarpophalangeal,
and proximal Interphalangeal joints. Also, rheumatoid arthritis is more likely
to be bilaterally symmetrical than is the case for Osteoarthritis.
9.6.3.5
Gouty Arthritis (GOW-tē)
9.6.3.5.1 Description
9.6.3.5.1.1 Uric acid (a
substance that gives urine its name) is a waste product produced during the
metabolism of nucleic acids. The person who suffers from gout either produces
excessive amounts of uric acid or is not able to excrete normal amounts. The
result is a buildup of uric acid in the blood. This excess acid then reacts
with sodium to form a salt called sodium urate. Crystals of this salt are
deposited in soft tissues. Typical sites are the kidneys, first metatarsal
Phalangeal joint and the cartilage of the ears and other joints.
9.6.3.5.2 Cause
9.6.3.5.2.1 In gouty
arthritis, sodium urate crystals are deposited in the soft tissues of the
joints. The crystals irritate the cartilage, causing inflammation, swelling,
and acute pain. Eventually, the crystals destroy all the joint tissues. If the
disorder is not treated, the ends of the articulating bones fuse and the joint
becomes immovable.
9.6.3.5.3 Treatment
9.6.3.5.3.1 Gouty arthritis
occurs primarily in middle-aged and older males. It is believed to be the cause
of 2 to 5 percent of all chronic joint diseases. Numerous studies indicate that
an abnormal gene sometimes causes gouty arthritis. As a result of this gene,
the body manufactures unusually large amounts of uric acid. Diet and
environmental factors such as stress and climate are also suspected causes of
gouty arthritis.
9.6.3.5.3.2 Although other
forms of arthritis cannot be treated with complete success, the treatment of
gouty arthritis with the use of various drugs has been quite effective. A
chemical called colchicines has been utilized periodically since the sixth
century to relieve the pain, swelling, and tissue destruction that occur during
attacks of gouty arthritis. This chemical is derived from the variety of crocus
plant from which the spice saffron is obtained. Other drugs, which either
inhibit uric acid production or assist in the elimination of excess uric acid
by the kidneys, are used to prevent further attacks. The drug allopurinal is
used for the treatment of gouty arthritis because it prevents the formation of
uric acid without interfering with nucleic acid synthesis. Rest and the use of
anti-inflammatory medication may obtain relief from an attack of gouty
arthritis.
9.6.4 Bursitis
Back Table
of Contents References
9.6.4.1
An acute chronic inflammation of a
bursa is called bursitis. The condition may be caused by trauma, by an acute or
chronic infection (including syphilis and tuberculosis), or by rheumatoid
arthritis. Repeated excessive friction often results in a bursitis with local
inflammation and the accumulation fo fluid. Bunions are frequently associated
with a friction bursitis over the head of the first metatarsal bone. Symptoms
include pain, swelling, tenderness, and the limitation of motion involving the
inflamed bursa. The prepatellar or subcutaneous Infrapatellar bursae may become
inflamed in individuals who spend a great deal of time kneeling. This bursitis
is usually called “housemaid’s knee” (“carpet layer’s knee”)
9.6.5 Dislocation
9.6.5.1
A dislocation, or luxation (luks-Ā-shun), is the displacement of a bone from a joint with tearing of ligaments,
tendons, and articular capsules. A partial or incomplete dislocation is called
a Subluxation. The most common dislocations are those involving a finger or
shoulder. Those of the mandible, elbow, knee, or hip are less common. Symptoms
include loss of motion, temporary paralysis of the involved joint, pain k,
swelling, and occasionally shock. A dislocation is usually caused by a blow or
fall., although unusual physical effort may lead to this condition.
9.6.6 Sprain and Strain
9.6.6.1
A sprain is the forcible wrenching or
twisting of a joint with partial rupture or other injury to its attachments
without luxation. It occurs when the attachments are stressed beyond their
normal capacity. There may be damage to the associated blood vessels, muscles,
tendons, ligaments, or nerves. A sprain is more serious than a strain, which is
the overstretching of a muscle. Severe sprains may be so painful that the joint
cannot be moved. There is considerable swelling and pain may occur owing to
underlying hemorrhage from ruptured blood vessels. The ankle joint is most
often sprained; the low back area is another frequent location for sprains.
10
Muscle Tissue
Back Table
of Contents References
10.1 Characteristics
10.2 Functions
10.3 Types
10.4 Skeletal
Muscle Tissue
10.4.1
10.4.2
Connective Tissue Components
10.4.3
Nerve and Blood Supply
10.4.4
Histology
10.4.4.1
Myofibers (Muscle Fibers or Cells)
10.4.4.1.1
Skeletal muscles consist of thousands of elongated cylindrical
cells called Myofibers or muscle fibers. The fibers lie parallel to one another
and range from 10 to 100 micro meters (µm) in diameter. Some muscle
fibers may reach lengths of 30 cm (12 inches) or more.
10.4.4.2
Sarcolemma (sarco=flesh; lemma=sheath)
10.4.4.2.1
Each muscle fiber is enveloped by a plasma membrane, which
contains the cytoplasm of the muscle cell called the Sarcoplasm.
10.4.4.3
Sarcoplasm
10.4.4.3.1
The cytoplasm to the muscle cell that contains many nuclei
(multinucleate) nestled close to the Sarcolemma. The Sarcoplasm also contains
myofibrils (Discussed below), mitochondrion, and enzymes.
10.4.4.4
Sarcoplasmic reticulum
(sar’-Kō-PLAZ-mik re-TIK-yoo-lum)
10.4.4.4.1
Membrane-enclosed tubules similar to smooth endoplasmic
reticulum (A network of channels running through the cytoplasm) (Substance
within plasma membrane) of other cells of the body which serves in
intracellular transportation, support storage, synthesis and packaging of
molecules.)
10.4.4.5
Terminal Cisterns
10.4.4.5.1
These are dilated sacs of sarcoplasmic reticulum, which form
ring like channels around the myofibrils.
10.4.4.6
Transverse tubles (T tubules)
10.4.4.6.1
These run transversely through the fiber and perpendicularly
to the sarcoplasmic reticulum and are extensions of the Sarcolemma that open to
the outside of the fiber.
10.4.4.7
Triad
10.4.4.7.1
Consists of a transverse tubule and terminal cisterns on either
side.
10.4.4.8
Myofibrils
10.4.4.8.1
Within the muscle cell there are 100-1000 cylindrical bundles
of muscle fiber 1 or 2 micro-meters (µm) in diameter composed of thin
and thick myofilaments.
10.4.4.9
Myofilaments
10.4.4.9.1
Thin Myofilaments
10.4.4.9.1.1
6 nm in diameter
10.4.4.9.2
Thick Myofilaments
10.4.4.9.2.1
16 nm in diameter
10.4.4.10 Sarcomeres
10.4.4.10.1
A compartment containing thin and thick Myofilaments
comprising the contractile unit bounded on either end by Z lines.
10.4.4.11 Z Lines
10.4.4.11.1
Dense material on either end of the sarcomere, which define
the contractile unit.
10.4.4.12 A (Anisotropic) Band
10.4.4.12.1
Represents the length of thick myofilaments, which are
darkened at either end by overlapping with the thin myofilaments attached to
the Z lines. The length of the darkening is increased with muscle contraction.
10.4.4.13 I (Isotropic) Band
10.4.4.13.1
Contains thin myofilaments only and has a Z line in the middle
representing the thin myofilaments of two adjacent contractile units. The
combination of alternating dark A bands and light I bands gives the muscle
fiber its striated (striped) appearance.
10.4.4.14 H Zone
10.4.4.14.1
A region in the center of the A band containing thick
myofilaments only.
10.4.4.15 M Line
10.4.4.15.1
The adjacent thick myofilaments are connected in the middle by
these fine threads.
10.4.4.16 Actin
10.4.4.16.1
Thin myofilaments are anchored to the Z lines and project in
both directions. Thin myofilaments are composed mostly of the protein actin.
The actin molecules are arranged in two single strands that entwine helically
and give the thin myofilaments their characteristic shape.
10.4.4.17 Myosin-Binding Site
10.4.4.17.1
The actin molecule has a myosin- binding site that interacts
with a cross bridge of a myosin molecule (discussed below).
10.4.4.18 Tropomyosin and Troponin (Tropomyosin-troponin Complex)
10.4.4.18.1
Proteins which help regulate muscle contraction
10.4.4.18.2
Tropomyosin
10.4.4.18.2.1
Arranged in strands that are loosely attached to the actin
helices.
10.4.4.18.3
Troponin
10.4.4.18.3.1
Located at regular intervals on the surface of Tropomyosin
with three subunits
10.4.4.18.3.2
Troponin I
10.4.4.18.3.2.1
Actin binding
10.4.4.18.3.3
Troponin C
10.4.4.18.3.3.1
Calcium binding
10.4.4.18.3.4
Troponin T
10.4.4.18.3.4.1
Tropomyosin binding
10.4.4.19 Myosin
10.4.4.19.1
Thick myofilaments are composed mostly of the protein myosin,
overlap the free ends of the thin myofilaments and occupy the A band of the
sarcomere.
10.4.4.19.2
The myosin molecule is golf club shaped, with tails (Golf club
handles) arranged parallel to one another, which form the shaft of the
myofilament. The heads of the golf
clubs project outward from the shaft and are arranged spirally of the surface
of the shaft.
10.4.4.20 Cross Bridges, Actin-Binding Site and ATP-Binding Site
10.4.4.20.1
The projecting heads of the myosin molecule are referred to as
cross bridges and contain an Actin-Binding Site and an ATP-Binding Site
10.5 Contraction
10.5.1
Sliding-Filament Theory
10.5.1.1
The myosin cross bridges pull on the
thin myofilaments causing them to slide inward toward the H zone. The lengths
of the thin and thick myofilaments do not change but the sarcomere shortens
evidenced by the reduced space between the Z lines. The myosin cross bridges of
the thick myofilaments connect with portions of actin of the thin myofilaments.
The myosin cross bridges move like the oars of a boat on the surface of the tin
myofilaments, and the thin and thick myofilaments slide past each other as the
cross bridges pull on, the thin myofilaments. The sliding of myofilaments and
shortening of sarcomeres causes the shortening of the muscle fibers.
10.5.2
Neuromuscular Junction
10.5.2.1
Neuron
10.5.2.1.1
Provides the stimulus for muscle contraction.
10.5.2.1.2
The thread-like process which delivers the nerve impulse is
called an axon can be 91 cm (3 feet) or more in length before attaching to a
muscle.
10.5.2.1.3
A bundle of axons from
many different neurons compose a nerve.
10.5.2.2
Motor Neuron
10.5.2.2.1
This is a neuron, which only stimulates muscles.
10.5.2.3
Motor End Plate
10.5.2.3.1
Upon entering the skeletal muscle the axon of a motor neuron
branches into axon terminals (telodendria) that come in contact with the
Sarcolemma of individual muscle cells.
10.5.2.3.2
This region of Sarcolemma axon contact is known as the motor
end plate.
10.5.2.4
Neuromuscular Junction (Myoneural
junction (MNJ))
10.5.2.4.1
Refers to both the axon terminal of a motor neuron along with
the motor end plate.
10.5.2.5
Synaptic end Bulbs
10.5.2.5.1
These are bulb like structures of the distal end of axon terminals
at the neuromuscular junction.
10.5.2.6
Synaptic Vesicles
10.5.2.6.1
Membrane enclosed sacs that store neurotransmitters.
10.5.2.7
Neurotransmitters
10.5.2.7.1
These chemicals determine whether an impulse is passed on to a
muscle (or gland or another nerve cell).
10.5.2.8
Synaptic Gutter (Trough)
10.5.2.8.1
This is the invaginated area of the Sarcolemma under the axon
terminal.
10.5.2.9
Synaptic Cleft
10.5.2.9.1
The space between the axon terminal and Sarcolemma
10.5.2.10 Subneural Clefts
10.5.2.10.1
These are folds of the Sarcolemma along the synaptic gutter.
10.5.2.10.2
This greatly increases the surface area of the synaptic gutter
allowing for increased numbers of receptor site molecules that are able to bond
to the neurotransmitter.
10.5.2.11 Acetylcholine (as’-ē-til-KŌ-lēn) (Ach)
10.5.2.11.1
Released from the synaptic vesicles (and possibly the
cytoplasm) when a nerve impulse (Action Potential) reaches the axon terminals
and synaptic end bulbs at the neuromuscular junction.
10.5.2.11.2
After release Ach diffuses across the synaptic cleft and
combines with receptor sites on the Sarcolemma of the muscle cell.
10.5.2.11.3
This combination alters the permeability of the Sarcolemma to
sodium (Na+) and potassium (Ka+) ions, and ultimately results in the
development of a muscle action potential that travels along the Sarcolemma and
is responsible for initiating the muscle contraction.
10.5.2.11.4
For the vast majority of muscle cells there is only one
neuromuscular junction for each cell located in the middle of the muscle cell.
10.5.2.11.5
The muscle action potential spreads from the center of the
Sarcolemma of the cell to the ends and through the transverse tubules.
10.5.2.11.6
This permits nearly simultaneous contraction of all sarcomeres
in the muscle cell as the action potential spreads deeply into the Sarcoplasm
by way of the transverse tubules of the Sarcolemma.
10.5.3
Motor Unit
10.5.3.1
The motor unit includes the motor
neuron and all the muscle fibers (cells) it stimulates.
10.5.3.2
150 muscle fibers may be stimulated by
just one motor neuron depending on the region of the body.
10.5.3.3
When one neuron of a motor unit is
stimulated this can result in the simultaneous contraction and relaxation of
all 150 muscle fibers of a motor unit that receive sufficient stimulation.
10.5.3.4
When precise movements are needed such
as for eye movements, these movements are carried out by the extrinsic
(external) eye muscles and there are fewer than 10 muscle fibers to each motor
unit.
10.5.3.5
Gross movements in muscles like the
gastrocnemius and biceps brachii may have as many as 200 muscle fibers in each
motor unit.
10.5.3.6
Recruitment (Motor Unit Summation)
10.5.3.6.1
Since stimulation of one motor neuron produces a contraction
in all the muscle fibers in a particular motor unit the total tension in a
muscle can be varied by adjusting the number of motor units that are activated.
10.5.3.6.2
The process of increasing the active number of motor units is
called recruitment and is determined by the demands that activity places on the
body.
10.5.3.6.3
Because not all motor units are active at the same time
(Neurons fire asynchronously=Some muscles inhibited others excited) this
prevents fatigue while maintaining contraction by allowing a brief rest for the
inactive units.
10.5.3.6.4
Thus contractions can
be maintained for long time periods with alternated smooth transitions between
active and inhibited motor units.
10.5.3.6.5
Continuous muscle tone and a state of partial contraction as
found in postural muscles
10.5.3.6.6
Recruitment helps produce smooth movements during muscle
contraction rather than a series of jerky movements.
10.5.4
Physiology of Contraction
10.5.4.1
A relaxed muscle has a low
concentration of calcium ions (Ca2 +) in the Sarcoplasm.
10.5.4.2
Calcium Ions are stored in the
sarcoplasmic reticulum.
10.5.4.3
A relaxed muscle has a high concentration
of ATP (Adenosine Triphosphate=(a-DEN-ō-sēn tri=FOS=fat)) and is
attached to the ATP-binding sites of the myosin cross bridges.
10.5.4.4
The binding of ATP to the myosin cross
bridges and the binding of the Tropomyosin-troponin complex to actin prevents
the myosin cross bridges from combining with actin of the thin myofilaments.
10.5.4.5
When a nerve impulse (nerve action
potential) reaches the synaptic end bulb of an axon terminal, a small amount of
calcium enters the synaptic end bulb, causing the synaptic vesicles (and
possibly the cytoplasm) to release Acetylcholine into the synaptic cleft.
10.5.4.6
It diffuses across the synaptic cleft
and combines with receptor sites on the Sarcolemma of the muscle Fiber directly
beneath the synaptic end bulb.
10.5.4.7
Acetylcholine-Gated Ion Channels
10.5.4.7.1
The combination of Acetylcholine with the receptor sites on
the Sarcolemma of the muscle fiber causes a change in the permeability of the
Sarcolemma in which membrane proteins called Acetylcholine-gated ion channels
open and permit a rapid influx of positive ions, mainly Na+.
10.5.4.8
Muscle Action Potential
10.5.4.8.1
The muscle action potential travels along the Sarcolemma and
then into the transverse tubules.
10.5.4.8.2
When the muscle action potentials conveyed over the transverse
tubules close to the sarcoplasmic reticulum, the action potential causes the
reticulum to release some of the calcium ions from storage into the Sarcoplasm
surrounding the myofilaments.
10.5.4.9
Calcium Release Channels
10.5.4.9.1
These are voltage-sensitive, tunnel-shaped proteins in the
sarcoplasmic reticulum which regulate the release of calcium from the
sarcoplasmic reticulum.
10.5.4.10 Troponin (Calcium-binding protein)
10.5.4.10.1
Undergoes structural changes when a portion of it combines
with calcium ions.
10.5.4.10.2
The Tropomyosin-troponin complex moves into a groove between
actin strands, thus exposing the myosin-binding sites on actin.
10.5.4.11 . ATP (Adenosine Triphosphate=(a-DEN-ō-sēn tri=FOS=fat))
10.5.4.11.1
Muscle contraction requires energy, as well as calcium ions.
The energy is supplied by ATP
10.5.4.11.2
ATP is attached to ATP-binding sites on myosin cross bridges.
10.5.4.11.3
Myosin cross bridges function as ATPase enzymes which can
split ATP into ADP (adenosine diphosphate) + P with the release of energy.
10.5.4.11.4
The muscle action potential stimulates a muscle fiber and the
myosin cross bridges functioning as ATPase enzymes, split ATP into ADP +
P. The ADP + P remain attached to their
binding sites on the myosin cross bridges, activated (energized) by the energy
from the splitting of ATP and combine with myosin-binding sites on actin.
10.5.4.12 Power Stroke
10.5.4.12.1
Once the activated myosin cross bridges are attached to the
myosin-binding sites on actin the activated myosin cross bridges change their
orientation.
10.5.4.12.2
The myosin cross bridge moves toward the H zone in the center
of the sarcomere and in so doing applies force to the thin actin myofilaments.
10.5.4.12.3
This movement of the myosin cross bridges is called the power
stroke and causes the thin actin myofilaments to slide past the thick myosin
myofilaments.
10.5.4.13 Detachment of Myosin Cross Bridge
10.5.4.13.1
Once the power stroke is complete, ATP combines with the ATP-binding
sites on the myosin cross bridges, resulting in the detachment of the myosin
cross bridge from actin.
10.5.4.14 Process Repeated
10.5.4.14.1
With the continued presence of a muscle action potential and
Ca ions the process repeats itself e.g. ATP is split and a given myosin cross
bridge then combines with another myosin-binding site further along the actin
strand.
10.5.4.14.2
The myosin cross bridges keep moving back and forth like the
cogs of a ratchet with each power stroke, moving the tin actin myofilaments
toward the H zone.
10.5.4.14.3
This continual movement applies the force that causes the Z
lines of a sarcomere to be drawn toward each other, and the sarcomere shortens.
10.5.4.14.4
The combined shortening of many sarcomeres results in global
muscle contraction of the entire motor unit.
10.5.4.15 Maximal Muscle Contraction
10.5.4.15.1
During maximal muscle contractions, the distance between Z
lines can be shortened to 50 % of the resting length.
10.5.4.16 Isometric Contraction
10.5.4.16.1
This cross bridge (contraction) does not always result in
shortening of the muscle fibers and muscle.
10.5.4.16.2
When the contraction does not result in muscle shortening, it
is called isometric contraction.
10.5.4.16.3
Using postural muscles to stand or sit is an example of an
isometric contraction.
10.5.4.17 Acetylcholinesterase (AchE)
10.5.4.17.1
Acetylcholine is rapidly destroyed by an enzyme called
Acetylcholinesterase (AchE). This allows the muscle fiber to go from a
contracted state back to a relaxed state.
10.5.4.17.2
This enzyme is found on the surfaces o the subneural clefts of
the Sarcolemma of muscle fibers.
10.5.4.17.3
As long as no further action potentials are generated there
will be no new release of Ach and this stops the generation of a muscle action
potential.
10.5.4.17.4
10.5.4.18 Calsequestrin and Calcium-ATPase
10.5.4.18.1
After the muscle action potential ends, the calcium ions are
actively transported from the troponin of the thin filaments in the Sarcoplasm
back into the sarcoplasmic reticulum for storage.
10.5.4.18.2
This is accomplished by two proteins called Calsequestrin and
Calcium-ATPase and involves an expenditure of some ATP, as the molecule is
broken down to release energy used to actively transport calcium ions into
storage in the sarcoplasmic reticulum.
10.5.4.19 Tropomyosin-Troponin Complex
10.5.4.19.1
Once calcium ions are removed from the Sarcoplasm, the
Tropomyosin-Troponin Complex is reattached to the actin strands, so that the
myosin-binding sites of actin become covered and myosin cross bridges separate
from actin and cannot reattach.
10.5.4.19.2
ATP is also required to detach the cross bridges.
10.5.4.20 Thin Myofilaments
10.5.4.20.1
Since the myosin cross bridges are broken, the thin
myofilaments slip back to their relaxed position.
10.5.4.21 ADP
10.5.4.21.1
After the muscle action potential ends, ADP is resynthesized
into ATP, which again attaches to the ATP-binding site of the myosin cross
bridge.
10.5.4.21.2
The sarcomeres are thereby returned to their resting lengths,
and the muscle fiber resumes its resting state.
10.5.4.21.3
During extensibility, sarcomere length can be increased by
about 20 percent of the resting length.
10.5.4.22 Summary
10.5.4.22.1
Nerve Impulse (Action Potential)
10.5.4.22.1.1
The action potential of the motor nerve causes synaptic vesicles
in motor axon synaptic end bulbs to release Acetylcholine (Ach).
10.5.4.22.2
Acetylcholine Diffusion and Muscle Action Potential
10.5.4.22.2.1
Acetylcholine diffuses across the synaptic cleft within the
neuromuscular junction and initiates a muscle action potential that spreads
over the surface of the Sarcolemma and transverse tubules.
10.5.4.22.3
Calcium Ionic Release
10.5.4.22.3.1
The muscle action potential enters the transverse tubules and
sarcoplasmic reticulum and stimulates the sarcoplasmic reticulum to release
calcium ions from storage into the Sarcoplasm.
10.5.4.22.4
Myosin-Binding Sites Exposed
10.5.4.22.4.1
Calcium ions combine with troponin, causing the
Tropomyosin-troponin complex to move, thus exposing the myosin-binding sites on
actin.
10.5.4.22.5
Power Stroke
10.5.4.22.5.1
When a muscle action potential stimulates a muscle fiber,
ATPase splits ATP into ADP + P and energy is released.
10.5.4.22.5.2
The released energy activates (energizes) myosin cross
bridges, which combine with the exposed myosin-binding sites on actin, and
apply force which causes the myosin cross bridges to move toward the H zone (Power
Stroke).
10.5.4.22.5.3
This movement results in the sliding of the thin myofilaments
past thick myofilaments.
10.5.4.22.6
Z Lines Narrow
10.5.4.22.6.1
The sliding draws the Z lines toward each other, the sarcomere
shortens, the muscle fibers contract and the muscle contracts.
10.5.4.22.7
Acetylcholine Inactivated
10.5.4.22.7.1
Acetylcholine is inactivated by Acetylcholinesterase (AchE).
As a result, Ach no longer has any effect at the neuromuscular junction.
10.5.4.22.8
Calcium Ions Reabsorbed
10.5.4.22.8.1
In the absence of further action potentials, calcium ions are
actively transported back into the sarcoplasmic reticulum by Calsequestrin and
calcium ATPase, using energy from ATP break down.
10.5.4.22.9
Tropomyosin-troponin complex Reattached
10.5.4.22.9.1
The low calcium concentration in the Sarcoplasm permits the
Tropomyosin-troponin complex to reattach to actin.
10.5.4.22.9.2
As a result, myosin-binding sites of actin become covered,
myosin cross bridges separate from actin.
10.5.4.22.9.3
ADP is resynthesized into ATP (which reattaches to the
ATP-binding site of the myosin cross bridge), and the thin myofilaments return
to their relaxed position.
10.5.4.22.10
Sarcomeres at Resting Length
10.5.4.22.10.1 Sarcomeres
return to their resting lengths, muscle fibers relax, and the muscle relaxes.
10.5.4.23 Clinical Application
10.5.4.23.1
Rigor Mortis
10.5.4.23.1.1
Following death, because of a lack of ATP, myosin cross
bridges remain attached to the actin myofilaments, thus preventing relaxation.
10.5.4.23.1.2
The myosin cross bridges need a constant stream of ATP to both
attach and detach to the actin myofilaments. Thus the lack of ATP makes
detachment impossible
10.5.4.23.1.3
The resulting condition in which muscles are in a state of rigidity
(cannot contract or stretch) is called rigor mortis (rigidity of death).
10.5.4.23.1.4
The time elapsing between death and the onset of rigor mortis
varies greatly among individuals.
10.5.4.23.1.5
Those who have had long, wasting illnesses undergo rigor
mortis more quickly.
10.5.4.23.1.6
Rigor mortis is not a permanent state that continues unabated
after death.
10.5.4.23.1.7
Depending on conditions, it lasts about 24 hours, then begins
to abate, and disappears after another 12 hours when tissues begin to
disintegrate, although a residual stiffness in the joints will remain.
10.5.4.23.2.1
Is caused by a defect in an enzyme, which breaks down glycogen
into glucose.
10.5.4.23.2.2
Since glycogen is stored in muscle tissue as a reserve energy
supply for muscle contraction it cannot be used for that purpose if it isn’t
converted to glucose by a defective enzyme.
10.5.4.23.2.3
The symptoms of this disease include, severe cramping and or
pain during exercise and muscle weakness later in life.
10.5.4.23.3.1
It serves to transport fatty acids across the cell membrane
and into the mitochondria, where these fatty acids are burned for energy. It
increases the rate of oxidation of fats in the liver, and this suggests that it
plays a role in improving energy generation from this angle as well.
10.5.5
Energy for Contraction and Relaxation
10.5.5.1
Phosphagen System
10.5.5.1.1
ATP
10.5.5.1.1.1
ATP is the immediate source of energy for muscle contraction
and is broken down by ATPase into ADP + P and energy synthesized by muscle
fibers as follows; ADP + P+ Energy=ATP.
10.5.5.1.1.2
The amount of ATP present in the muscle fibers is sufficient
to maintain muscle contraction during vigorous exercise for 5 to 6 seconds.
10.5.5.1.2
Phosphocreatine (fos’fō-KRĒ-a-tin)
10.5.5.1.2.1
Skeletal muscle fibers contain this high-energy molecule,
which is used to generate ATP rapidly.
10.5.5.1.2.2
There are about 2 to three times greater amounts of
Phosphocreatine than ATP in the muscle fiber.
10.5.5.1.2.3
Phosphocreatine breaks down into creatine and phosphate and
produces large amounts of energy as follows; Phosphocreatine=Creatine +
Phosphate + Energy.
10.5.5.1.2.4
Some of the released energy is used to convert ADP to ATP and
takes place in a fraction of a second to provide enough energy for muscles to
contract maximally for about 15 seconds.
10.5.5.1.2.5
This energy system is used for maximal short bursts of energy
and allows time for the rate of two metabolic processes, glycolysis and
cellular respiration to be increased.
10.5.5.2
Glycogen-Lactic Acid System
10.5.5.2.1
Glycolysis
10.5.5.2.1.1
After Phosphocreatine depleted, then the source of energy is
glucose, which is derived from the breakdown of glycogen (stored glucose),
which is stored in the muscles, liver and found in the blood.
10.5.5.2.1.2
Glycogen breakdown is accomplished with calcium ions and a
calcium-binding protein called calmodulin.
10.5.5.2.1.3
Once glucose is produced by this breakdown, each molecule of
glucose is split into two molecules of pyruvic acid, a process called
glycolysis.
10.5.5.2.1.4
This process releases energy which is used to form ATP and
because glycolysis does not require oxygen it is referred to as an anaerobic
process summarized as follows; 1 Glucose=2 Pyruvic acid + Energy (ATP).
10.5.5.2.2
Glycogen-Lactic Acid System
10.5.5.2.2.1
When there is not sufficient oxygen for the complete
catabolism of pyruvic acid, it is converted to lactic acid, some of which
diffuses out of the muscle fibers and eventually into blood.
10.5.5.2.2.2
The production of lactic acid in this way releases energy from
glucose that can be used to produce ATP, and it occurs anaerobically providing
sufficient energy for about 30-40 seconds fo maximal muscle activity.
10.5.5.3
Aerobic System
10.5.5.3.1
Cellular Respiration
10.5.5.3.1.1
When sufficient oxygen is present, the pyruvic acid formed by
glycolysis enters the mitochondria of muscle fibers, where it is completely
catabolized to carbon dioxide and water in the metabolic process called
cellular respiration.
10.5.5.3.1.2
This is considered an aerobic process since it requires
oxygen.
10.5.5.3.1.3
The complete catabolism of pyruvic acid also yields energy
that is used to generate most of a muscle fiber’s ATP: Pyruvic Acid + O2
=CO2 + H2O + Energy (ATP).
10.5.5.3.1.4
This process will continue as long as nutrients (fatty acids
(from Fats), amino acids (from proteins), and glucose derived from glycogen
breakdown or delivered to the muscle by way of blood) and adequate oxygen last.
10.5.5.4
Summary
10.5.5.4.1
Phosphagen System
10.5.5.4.1.1
15 seconds muscular activity=100-meter dash
10.5.5.4.2
Glycogen-lactic Acid System
10.5.5.4.2.1
30-40 seconds muscular activity=400-meter dash
10.5.5.4.3
Aerobic System
10.5.5.4.3.1
Prolonged activity as long as sufficient oxygen and nutrients
are available=Jogging
10.5.6
All-or-None Principle
10.5.7
Muscle Tension
10.5.8
Muscle Tone
10.5.9
Muscular Atrophy and Hypertrophy
10.5.10
Types of Skeletal Muscle Fibers
10.6 Cardiac
Muscle Tissue
10.7 Smooth
Muscle Tissue
10.8 Regeneration
of Muscle Tissue
10.9 Homeostasis
10.10
Aging and Muscle Tissue
10.11
Developmental Anatomy of the Muscular
System
10.11.1
Back Table
of Contents References
Link To Muscular System Chapter
12.1 Introduction
12.1.1
12.2 Organization
12.2.1
12.3 Histology
12.3.1
12.3.2
Neuroglia
12.3.2.1
12.3.3
Neurons
12.3.3.1
12.3.3.2
Structure
12.3.3.2.1
12.3.3.3
Structural Variation
12.3.3.3.1
12.3.3.4
Classification
12.3.3.4.1
12.4 Physiology
12.4.1
12.4.2
Nerve Impulse
12.4.2.1
12.4.2.2
Membrane Potentials
12.4.2.2.1
12.4.2.3
Excitability
12.4.2.3.1
12.4.2.4
All-or-None Principle
12.4.2.4.1
12.4.2.5
Saltatory Conduction
12.4.2.5.1
12.4.2.6
Speed of Nerve Impulses
12.4.2.6.1
12.4.3
Conduction across Synapses
12.4.3.1
12.4.3.2
Excitatory Transmission
12.4.3.2.1
12.4.3.3
Inhibitory Transmission
12.4.3.3.1
12.4.3.4
Integration at Synapses
12.4.3.4.1
12.4.4
Neurotransmitters
12.4.4.1
12.4.5
Regeneration
12.4.5.1
12.5 Organization
of Neurons
12.5.1
12.6 Neurons and Supporting
Cells Human Physiology Fox 150-151
12.6.1
Neurons produce and conduct
electrochemical impulses and along with supporting cells comprise the nervous
system.[1]
12.6.2
The nervous system is composed of neurons, which produce and conduct
electrochemical impulses, and supporting cells, which assist the functions of
neurons. Neurons are classified functionally and structurally; the various
types of supporting cells perform specialized functions.
12.6.3
The nervous system is divided into the central nervous system (CNS),
which includes the brain and spinal cord, and the peripheral nervous system
(PNS), which includes the cranial nerves arising from the brain and the spinal
nerves arising from the spinal cord.
12.6.4
The nervous system is composed of only two principal types of cells-neurons
and supporting cells. Neurons are the basic structural and functional units of
the nervous system. They are specialized to respond to physical and chemical
stimuli, conduct electrochemical impulses, and release chemical regulators.
Through these activities, neurons enable the perception of sensory stimuli,
learning, memory, and the control of muscles and glands. Most neurons cannot
divide by mitosis, although many can regenerate a severed portion or sprout
small new branches under certain conditions.
12.6.5
Supporting cells aid the functions of neurons and are about five times
more abundant than neurons. In the CNS, supporting cells are collectively
called neuroglia, or simply glial cells (glia = glue). Unlike neurons, glial
cells retain limited mitotic abilities (brain tumors that occur in adults are
usually composed of glial cells rather than neurons).
12.6.6
Neurons
12.6.6.1 Although neurons vary
considerably in size and shape, they generally have three principal regions:
(1) a cell body, (2) dendrites, and (3) an axon (Illustration # 2). Dendrites and axons can
be referred to generically as processes or
extensions from the Cell body (Neuron).
12.6.6.2
The cell body, or perikaryon (peri=
around; karyon = nucleus), is the
enlarged portion of the neuron that contains the nucleus. It is the
"nutritional center" of the neuron where macromolecules are produced.
The cell body also contains densely staining areas of rough endoplasmic
reticulum known as Nissl bodies that
are not found in the dendrites or axon. The cell bodies within the CNS are
frequently clustered into groups called nuclei
(not to be confused with the nucleus of a cell). Cell bodies in the PNS
usually occur in clusters called ganglia (Illustration # 2).
12.6.6.3
Dendrites
12.6.6.3.1
Dendrites (dendron = tree branch) are thin, branched
processes that extend from the cytoplasm of the cell body. Dendrites provide a
receptive area that transmits electrical impulses to the cell body.
12.6.6.4
Axon
12.6.6.4.1
The
axon is a longer process that conducts impulses away from the cell body. Axons
vary in length from only a millimeter long to up to a meter or more (for those
that extend from the CNS to the foot). The origin of the 'axon near the cell
body is an expanded region called the axon hillock; it is here that nerve impulses originate. Side branches
called axon collaterals may extend from the axon.
12.6.6.5
Proteins and other molecules are transported through the axon at faster
rates than could be achieved by simple diffusion. This rapid movement is produced
by two different mechanisms: axoplasmic flowAxoplasmic flow and axonal transport
(table 7.2).
12.6.6.6
Axoplasmic flow
12.6.6.6.1
Axoplasmic
flow, the slower of the two, results from rhythmic waves of contraction that push
the cytoplasm from the axon hillock to the nerve endings. Axonal
transport, which employs rnicrotubules and is more rapid and more selective, may
occur in a reverse (retrograde) direction as well as in a forward (orthograde)
direction. Indeed, retrograde transport may be responsible for the movement of
herpes virus, rabies virus, and tetanus toxin from the nerve terminals into
cell bodies.
12.6.7
Test Your
Knowledge Section Test
Section 2.2.2 Test 3
13.1
13.2
Grouping of Neural Tissue Principles of
A&P 355
13.2.1
Introduction
13.2.1.1
White matter consists of myelinated
nerve fibers such as dendrites or axons, which are grouped together in tracks
and supported by glial cells. Gray
matter consists of unmyelinated axons or dendrites and nerve cell bodies. The
absence of myelin in these areas accounts for their gray color. A tract is a
bundle of nerve fibers consisting of dendrites and axons that run long
distances up and down the spinal cord and connect parts of the brain. For our purposes, these tracks in the spinal
cord consist of ascending sensory fibers or descending motor fibers. The spinal cord itself consists of both gray
and white matter. The horns of the
spinal cord consist of gray matter in the form of an H shape. If the H is situated
with the top toward the anterior portion of the body the top of H shape is
called the anterior Gray Horn and the bottom of the H shape is called the
posterior Gray Horn. On the outside of
the H is the lateral Gray Horn. The
crossbar of the H is situated in the center of the spinal cord. The white columns are divided into anterior
and posterior white columns and lateral white columns. Down the center of the spinal cord is a
division much as in the brain and is called a sulcus. The two halves to the spinal cord are connected in the center,
which is also, where the center of the H is situated. The crossbar of the H is
known, as the gray commissure. At the
center of the gray commissure is the central canal, which is contiguous with
the fourth ventricle of the brain.
Anterior to the gray commissure is the anterior white commissure which
connects the two half’s of the spinal cord.
13.3
Spinal
Cord
13.3.1
Protection
and Coverings
13.3.1.1
Vertebral
Canal
13.3.1.1.1
13.3.1.2
Meninges Principles of A&P 355
13.3.1.2.1
The spinal cord successive layers of covering (meninges) with
the outermost layer are known as the dura mater (tough mother). The meninges cover and protect the spinal
cord and run continuously around the spinal cord and brain. Between the dura mater and the vertebral
canal is the epidural space, which is filled with fat, connective tissue, and
blood vessels. This tissue helps pad the spinal cord from injury. It is in the
epidural space at the level of the second lumbar vertebrae that doctors will
inject an anesthetic for both the pain for an irritated nerve or as a saddle
block for childbirth. The second
innermost covering of the spinal cord is the arachnoid (spider like). This web like structure is also continuous
with the arachnoid of the brain. The
subdural space is in between the arachnoid and the dura mater. The innermost covering of the spinal cord is
called the pia mater (delicate mother) it connects with the spinal cord and
although it is fibrous, it is transparent.
It contains numerous blood vessels and nerves. The space between the arachnoid and the pia mater is the
subarachnoid space. This space contains
the cerebral spinal fluid. Inflammation
of the meninges is known as meningitis.
The most common form of meningitis is inflammation of the arachnoid and
pia mater and is known as leptomeningitis.
13.3.2
General Features Principles of A&P 355
13.3.2.1
The spinal cord is about 1 1/2 ft.
long and begins as a continuation of the medulla oblongata, which is the
inferior part of the brain's stem, and extends from the foramen magnum of the
occipital bone to the upper border of the 2nd lumbar vertebrae. It is cylindrical in shape and slightly
flattened from the anterior to the posterior.
The spinal cord is about 1 in. in circumference in the mid thoracic
region but is enlarged in both the cervical (cervical enlargement) and the
lumbar region (lumbar enlargement). The
spinal cord forms an unbroken tube from its exit at the foramen
magnum and the cranial cavity to the filum terminale and its connection at
the coccyx. Its protective coverings are called meninges. The meninges of the spinal cord are
continuous with the meninges of the brain. The filum terminale is a non-nervous
fibrous tissue composed mostly of pia mater. It attaches to the end of the
spinal cord at the conus medullaris, which is located at the intervertebral
disc between the first and 2nd lumbar vertebrae. The filum terminale then attaches to the coccyx. The spinal cord is then securely anchored at
two places, at both the cranium and the coccyx. The spinal cord is also
attached along its entire route to the vertebral column, by denticulate
ligaments, which act as shock absorbers in protecting the cord from injury.
13.3.3
Structure
in Cross Section
13.3.3.1
13.3.4
Functions
13.3.4.1
13.3.4.2
Impulse
Conduction
13.3.4.2.1
13.3.4.3
Reflex
Center
13.3.4.3.1
The second
principal function of the spinal cord is to serve as a center for some reflex
actions. Spinal nerves are the paths of
communication between his spinal cord tracts and the periphery. Each pair of spinal nerves is connected to a
segment of the cord by two points of attachment called roots. The posterior or dorsal (sensory) root
contains sensory nerve fibers only and conducts nerve impulses from the
periphery to the spinal cord. These
fibers extend into the posterior (dorsal) Gray horn. Each dorsal root also has a swelling, the posterior or dorsal
(sensory) root ganglion that contains the cell bodies of the sensory neurons
from the periphery. The other point of
attachment of a spinal nerve to the cord is the anterior or ventral (motor)
root. It contains motor neuron axons
only and conducts impulses from spinal cord to the periphery.
13.3.4.3.2
The cell
bodies of the motor neurons are located in the gray matter of the cord. If the motor impulse supplies the skeletal
muscle, the cell bodies are located in the anterior (ventral) Gray horn. If, however, the motor nerve impulse
supplies smooth muscle, cardiac muscle, or a gland through the autonomic
nervous system the cell bodies are located in the lateral gray horn.
13.3.4.4
Reflex
Arc and Homeostasis
13.3.4.4.1
Introduction
13.3.4.4.1.1
The path a nerve impulse follows from its origin in the
dendrites or cell body of a neuron in one part of the body to its termination
elsewhere in the body is called a conduction pathway. All conduction pathways consist of circuits of neurons. One pathway is known as a reflex arc, the
functional unit of the nervous system.
A reflex arc contains two or more types of neurons over which nerve
impulses are conducted from a receptor to the brain or spinal cord by way of
sensory neurons and then to an effector by way of motor neurons. The basic components of a reflex arc are as
follows;
13.3.4.4.1.1.1
Receptor
13.3.4.4.1.1.1.1
The distal end of a dendrite or a sensory structure associated
with the distal end of a dendrite. Its role in the reflex arc is to respond to
a specific change in the internal or external environment by initiating a nerve
impulse in a sensory neuron by way of a receptor potential (a local
depolarization of the receptor cell membrane).
13.3.4.4.1.1.2
Sensory neurons
13.3.4.4.1.1.2.1
Passes the nerve impulse from the receptor to its axonal
termination in the central nervous system.
13.3.4.4.1.1.3
Center
13.3.4.4.1.1.3.1
A region in the central nervous system where an incoming
sensory impulse generates an outgoing motor impulse. In the center, the impulse may be inhibited, transmitted, or
rerouted. In the center of some reflex
arcs, the sensory neuron directly generates the impulse in the motor
neuron. The center usually contains one
or more association neurons between the sensory neuron and the motor neuron
leading to a muscle or gland.
13.3.4.4.1.1.4
Motor neuron
13.3.4.4.1.1.4.1
Transmits the impulse generated by the sensory or association
neuron in the center to the effector organ of the body that will respond, such
as a muscle or gland.
13.3.4.4.1.1.5
Effector
13.3.4.4.1.1.5.1
The organ of the body that responds to the motor nerve
impulse. This response is called a
reflex and involves either an increase or decrease in muscle contraction or an
increase or decrease in secretion by glands.
13.3.4.4.2
Reflexes
13.3.4.4.2.1
These are fast responses to certain changes (stimuli) in the
internal or external environment that allow the body to maintain
homeostasis. Reflexes are associated
not only was skeletal muscle contraction but also with body functions such as
heart rate, respiration, digestion, urination, and defecation, which involve
cardiac and smooth muscle and glands.
Reflexes carried out by the spinal cord alone are called spinal
reflexes. Reflexes that result in
contraction of skeletal muscles are known as somatic reflexes. Reflexes that involve brain centers cranial
nerves are called cranial reflexes.
Those that cause the contraction of smooth are cardiac muscle or
secretion by glands are visceral (autonomic) reflexes. Our concern at this point is to examine a
few somatic spinal reflexes: the
stretch reflex, tendon reflex, flexor reflex and crossed extensor reflex.
13.3.4.4.3
Physiology of the Stretch Reflex
13.3.4.4.3.1
The stretch (tendon jerk) reflex is based on a two neuron or
monosynaptic reflex arc. Only two types
of neurons (one sensory and one motor) are involved, and there's only one
synapse in the pathway. This reflex
results in the contraction of a muscle when it is stretched suddenly. Slight stretching of the muscle stimulates
receptors in the muscle called muscle spindles. The spindles monitor changes in the length of the muscle by
responding to the rate and degree of change in length. Once the spindle is stimulated (depolarized
to threshold), a nerve impulse is sent along a sensory neuron through the
posterior root of the spinal nerves to the spinal cord. The sensory neuron
synapses with a motor neuron in the anterior gray horn. The sensory neuron
generates an impulse at the synapse that is transmitted along the motor neuron.
The motor neuron in the anterior root of the spinal nerve terminates in a
skeletal muscle. Once the impulse reaches the stretched muscle, a muscle action
potential is generated and the muscle contracts. Thus, the stretch is
counteracted by contraction, which shortens the muscle that had been stretched.
13.3.4.4.3.2
Since the sensory nerve impulse enters the spinal cord on the
same side that the motor nerve impulse leaves the spinal cord, the reflex arc
is called an ipsilateral (ip’si-LAT-er-al) reflex arc. All monosynaptic reflex
arcs are ipsilateral as are deep tendon reflexes (discussed shortly).
13.3.4.4.3.3
The stretch reflex is essential in maintaining muscle tone and
is important for muscle functions during exercise. It also helps prevent injury
from overstretching of muscles. Moreover, it is the basis for several tests
used in neurological examinations. One such reflex is the patellar reflex (knee
jerk). This reflex involves extension of the leg by contraction of the
quadriceps femoris muscle in response to tapping the patellar ligament. When
the patellar ligament below the patella is tapped (stimulus), receptors (muscle
spindles) in the quadriceps femoris muscle sensitive to changes in muscle
length are stimulated because tapping the tendon of the muscle causes a rapid
lengthening of the muscle. Sensory impulses from the receptors are sent to the
spinal cord, and the returning motor nerve impulse results in generation of a
muscle action potential that causes contraction of the muscle and extension of
the leg at the knee, or a knee jerk as the muscle shortens during contraction.
13.3.4.4.3.4
Although the stretch reflex pathway contains only two types of
neurons, other neurons are involved in the pathway in various ways. For
example, the sensory neuron from the muscle spindle that enters the spinal cord
also synapses with an inhibitory association neuron that, in turn, synapses
with a motor neuron that controls antagonistic muscles. The inhibitory
association neuron inhibits the motor neurons that normally send excitatory
impulses to antagonistic muscles. Thus, during the stretch reflex when the
stretched muscle is counteracted by contraction, antagonistic muscles that
oppose the contraction are inhibited. This phenomenon, by which action
potentials stimulate contraction of one muscle and simultaneously inhibit
contraction of antagonistic muscles, is called reciprocal innervation. It
avoids conflict between prime movers and antagonists and is vital in
coordinating body movements. Since skeletal muscles act in groups rather than
alone and that each muscle in the group (agonist, antagonist, synergist,
fixator) has a specific role in bringing about the movement.
13.3.4.4.3.5
The sensory neuron from the muscle spindle also synapses with
neurons that relay impulses to the brain by way of sensory tracts. In this way,
the brain is provided with information about the state of stretch or
contraction of skeletal muscles that enables it to coordinate muscular
movements and posture.
13.3.4.4.4
Physiology of the Tendon Reflex
13.3.4.4.4.1
Reflexes other than the stretch reflex involve association
neurons in addition to sensory and motor neurons. Since more than two types of
neurons are involved, there is more than one type of synapse, and thus these
reflexes are polysynaptic reflex arcs. One example of a reflex based on a
polysynaptic reflex arc is the tendon reflex. The tendon reflex, like the
stretch reflex, is also ipsilateral.
13.3.4.4.4.2
Just as the stretch reflex operates as a feedback mechanism to
control muscle length, the tendon reflex operates as a feedback mechanism to
control muscle tension by protecting tendons and their associated muscles from
excessive tension. The receptors for this reflex are called tendon organs or
Golgi tendon organs. Tendon organs lie within muscle tendons near the junction
of a tendon and a muscle. Whereas muscle spindles are sensitive to changes in
muscle length, tendon organs detect and respond to changes in muscle tension
caused by passive stretch or muscular contraction.
13.3.4.4.4.3
When an increase in tension is applied to a tendon, the tendon
organ is stimulated (depolarized to threshold) and nerve impulses are generated
and transmitted to the spinal cord via a sensory neuron. Within the spinal
cord, the sensory neuron synapses with an inhibitory association neuron, which,
in turn, synapses with and hyperpolarizes (inhibits) a motor neuron that
innervates the muscle associated with the tendon organ. Thus, as tension on the
tendon organ increases, the frequency of inhibitory impulses increases, and the
inhibition of the motor neurons to the muscle developing excess tension causes
relaxation of the muscle. In this way, the tendon reflex protects the tendon
and muscle from damage from excessive tension. Thus, the tendon reflex is
protective.
13.3.4.4.4.4
The sensory neuron from the tendon organ also synapses with a
stimulatory association neuron in the spinal cord. The stimulatory association
neuron, in turn, synapses with motor neurons controlling antagonistic muscles.
Thus, whereas the tendon reflex brings about relaxation of the muscle
containing the tendon organ, it also brings about contraction of the antagonistic
muscles. This is another example of reciprocal innervation. The sensory neuron
also synapses with neurons that relay nerve impulses to the brain by way of
sensory tracts, thus informing the brain about the state of muscle tension
throughout the body.
13.3.4.4.5
Physiology of the Flexor Reflex and Crossed Extensor Reflex
13.3.4.5
Reflexes
and Diagnosis
13.3.4.5.1
13.4
Spinal
Nerves
13.4.1
Names
13.4.1.1
13.4.2
Composition
and Coverings
13.4.2.1
13.4.3
Distribution
13.4.3.1
Branches
13.4.3.1.1
13.4.3.2
Plexuses
13.4.3.2.1
13.4.3.2.2
Cervical Plexus
13.4.3.2.3
Brachial Plexus
13.4.3.2.4
Lumbar Plexus
13.4.3.2.5
Sacral Plexus
13.4.3.2.5.1
Sacral Plexus Nerves
13.4.3.2.5.1.1
Sciatic
13.4.3.2.5.1.1.1
Its nerve roots are L4-S3.. Composed of two nerves: tibial and
common peroneal, bound together by common sheath of connective tissue.
13.4.3.2.5.1.1.2
It splits into its two divisions, usually at knee.
13.4.3.2.5.1.1.3
As the sciatic nerve descends through thigh, it sends branches
to hamstring muscles (biceps femoris, semitendinousus, semimembranosus) and
adductor magnus.
1.1.1.1.1.1.1.1.1
Tibial (medial popliteal)
1.1.1.1.1.1.1.1.2 Its nerve roots are
L4-S3. Innervates gastrocnemius, Plantaris, Soleus, Popliteus, Tibialis
posterior, flexor digitorum longus and flexor hallucis longus muscles.
1.1.1.1.1.1.1.1.3 Branches of tibial nerve in foot are medial
plantar nerve and lateral plantar nerve.
1.1.1.1.1.1.1.1.4
Medial Plantar
1.1.1.1.1.1.1.1.5 Abductor hallucis,
flexor digitorum brevis, and flexor hallucis brevis muscles, skin over medial
two-thirds of plantar surface of foot.
1.1.1.1.1.1.1.1.6
Lateral Plantar
1.1.1.1.1.1.1.1.7 Remaining muscles of
foot not supplied by medial plantar nerve; skin over lateral third of plantar
surface of foot.
1.1.1.1.1.1.1.1.8
Common Peroneal (lateral popliteal)
1.1.1.1.1.1.1.1.9 Superficial Peroneal
1.1.1.1.1.1.1.1.10
Peroneus longus and Peroneus brevis muscles; skin over distal
third of anterior aspect of leg and dorsum of foot.
1.1.1.1.1.1.1.1.11
Deep Peroneal
1.1.1.1.1.1.1.1.12
Tibialis anterior, extensor hallucis longus, Peroneus Tertius,
and extensor digitorum longus and brevis muscles; skin over great and second
toes.
13.4.3.3
Intercostal
(Thoracic) Nerves
13.4.3.3.1
13.4.4
Dermatomes
13.4.4.1
13.4.4.2 Dermal Segmentation Ciba 55
13.4.4.2.1
Sensations
from the outside world are carried to the consciousness by the spinal and some
cranial nerves to the brain. They travel over the posterior roots; their
further detailed course is for the moment " not of interest. All the cell
bodies are situated in ganglia outside of the central nervous system proper;
they have a peripheral branch going to the "sense organ and a central
branch going to the spinal cord or the brain.
13.4.4.2.2
We
include here the fibers carrying hot and cold, pain and touch. Vibration sense
and position sense are probably complicated sensations involving several of the
primitive sensations, including the proprioceptive sensations, which in the
spinal cord go also into the ganglia, but in the head region end in the
mesencephalic nucleus of the trigeminal nerve.
13.4.4.2.3
In the
head, sensations are mediated by the trigeminus and in a small region behind
the external auditory meatus by the facial and the vagus nerves. In the body,
the various spinal nerves take over. These are largely arranged metamerically,
that is to say, one segment lies behind the other in an orderly sequence.
However, these sequences are interrupted in the arm and the leg regions where
several segments have been carried out to the periphery of the limb and are no
longer represented near the axis of the body. Hence, on the ventral side of the
body, C5 borders on TI, and LI borders next to S2.
13.4.4.2.4
13.4.4.2.5
Two
other important points should be realized: The segmentations given in almost
all diagrams are based on pain, but the borders for the reception of pain are
not the same as those for the reception of, say, touch, which generally
involves a somewhat narrower sphere. The second point is that the different
nerves overlap to some extent, so that frequently no more than a hypesthesia
develops after one root has been cut.
13.4.4.2.6
If one
is familiar with the cutaneous distribution of various nerve roots; it is
possible to localize the site and level of any pathologic disturbance.
13.4.4.2.7
A
chart outlining the exact sensory dermal segments serves as a good reference,
but it is valuable to remember some surface landmarks which will serve as a
general guide to localization: Illustration #4 Illustration #4b
13.4.4.2.8
Cervical
five sensory roots supply the clavicular region.
13.4.4.2.9
Cervical
five and six sensory roots supply the deltoid region.
13.4.4.2.10
Thoracic
four sensory roots supply the nipple area.
13.4.4.2.11
Thoracic
10 sensory roots supply the umbilicus.
13.4.4.2.12
The
groin region is supplied by thoracic I2 sensory root. .
13.4.4.2.13
Cervical
5,6 and 7 sensory roots supply the lateral aspect of the arm.
13.4.4.2.14
The
inner aspect of the arm is supplied by cervical eight and thoracic one sensory
roots.
13.4.4.2.15
Lumbar
L1, 2, 3 and 4 sensory roots supply the inner and anterior surfaces of the
thigh from above down.
13.4.4.2.16
Lumbar
5 and sacral I and 2 sensory roots supply the outer and posterior surfaces of
the thigh. "
13.4.4.2.17
Sacral
2, 3, 4 and 5 sensory roots supply the perineum.
13.4.4.2.18
The
hand is supplied from the radial to the ulnar borders by cervical 6, 7 and 8
sensory roots. Cervical 6 supplies the thumb, cervical 7 the middle of the
hand, including the index and middle fingers, and cervical 8 the ulnar border,
including the ring and little fingers.
13.4.4.2.19
Sacral
1, lumbar 5 and lumbar 4 sensory roots supply the foot from its lateral to its
medial surface.
13.4.4.2.20
NOTE: Some
discrepancies exist in the definition of the dermal segments as published by
different authors, but the chart produced here is based essentially on the work
of Dr. J. Jay Keegan, University of Nebraska C[2]ollege of Medicine. We herewith express our appreciation for his
contributions and personal communication. [3] [4] [5]
13.4.4.3
Dermatome??
13.4.4.3.1
It is something of a miracle, that we evolve into what we are
today from such humble beginnings. From
just a few cells in the womb of our mother, we become what we are today. The Fetal stem cells form the organs, bones,
muscles, nerves and fascia. The
specialized set of cells that form the fascia including the skin is called a
dermatome. The word dermatome has also
come to mean the area of the skin supplied by the dorsal RAMI of the spinal
nerves. Each dermatome is named after a spinal level from which the nerve
originates. For example, the L 1
dermatome is supplied by the first lumbar nerve. The L5 dermatome is supplied by the 5th lumbar nerve. These sensory nerves allow us to feel both
the caressing touch of a loved one, and the burn of a frying pan.
13.4.4.3.2
Our
muscles, bones & skin form when we are still in the womb.
13.4.4.3.3
The
dermatome is the name for a specialized set of cells in the developing fetus, which
forms the connective tissues, including the dermis or skin. Illustration # 4
13.4.4.3.4
–The skin over
the entire body is supplied segmentally by spinal nerves.
13.4.4.3.5
The skin
segment supplied by the dorsal root of a spinal nerve is also called a
dermatome.
13.4.5
Arteries of the Spinal Cord Ciba 53 Illustration # 5
13.4.5.1 The spinal cord derives
its blood supply from the vertebral artery and from a series of spinal rami,
which enter the intervertebral foramina at successive levels.
13.4.5.2 The posterior spinal
artery is a branch of the vertebral artery, which begins near the lateral
margin of the medulla oblongata and descends on the dorsolateral surface of the
spinal cord posterior to the spinal roots. In its downward course to the cauda,
equina the posterior spinal artery receives a succession of small arterial
branches, which enter the spinal canal through the intervertebral foramina.
These vessels and their branches anastomose freely around the posterior roots
and with the corresponding vessels on the opposite side, dipping also into the
substance of the spinal cord, and in the midline form the posterior central
artery.
13.4.5.3 The anterior spinal
artery is formed by the union of two branches from the terminal portion of the
vertebral artery at the level of the foramen magnum. The artery descends as a
single trunk on the anterior aspect of the spinal cord to the conus medullaris,
then continues along the cauda equina and ends as a fine arteriole accompanying
the filum terminale. At successive levels spinal branches entering through the
intervertebral foramina, reinforce it. Along its course, small twigs from this
artery enter the sub- stance of the spinal cord and in the anterior median
fissure these form the anterior central artery.
13.4.5.4 The spinal branches arise
at various levels from the sacral, iliolumbar, intercostal, inferior thyroid
and vertebral arteries, which enter the spinal canal through the intervertebral
foramina. Each spinal branch divides into two rami.
13.4.5.5 A peripheral ramus, which
after entering the spinal canal divides into an ascending and descending
branch. It then anastomoses with the one above and below to form two lateral
chains on the posterior surfaces of the vertebral bodies near the junction of
the pedicles.
13.4.5.6 (2) A central ramus,
which supplies the spinal cord and its membranes by dividing into anterior and
posterior arteries, which anastomose with the anterior and posterior arteries
of the spinal cord.
13.4.6
Venous Drainage of Spinal Cord and Vertebral Column Illustration # 6
Ciba 54
13.4.6.1 Outside and inside the
vertebral canal, running along the entire length, is a series of venous
plexuses which freely join with each other and end in intervertebral veins.
13.4.6.2
Two groups of venous plexuses are found on the outside of the vertebral
canal: (I) the anterior group lies in front of the vertebral bodies. This group
receives some venous tributaries from vertebral bodies and communicates with
the basivertebral and intervertebral veins, and (2) the posterior group, which
forms a network of venous plexuses spreading over the spinous processes,
laminae, facets and adjacent deep musculature. In the cervical region, these
veins communicate with the deep cervical, occipital and cerebral veins.
13.4.6.3 The venous plexuses on
the inside of the vertebral canal lie between the dura and inner vertebral
surfaces. These veins receive tributaries from the adjacent bony structures and
the spinal cord. Although they form a close network, running vertically within
the spinal canal, they may be subdivided as follows.
13.4.6.4 A pair of anterior
internal venous plexuses which lie on either side of the posterior longitudinal
ligament and into which basivertebral veins empty.
13.4.6.5 (2) A single posterior
internal venous plexus which lies anterior to, and on either side of, the vertebral
arches and ligamentum flavum, and anastomose with the posterior external veins.
13.4.6.6 These plexuses form
almost a series of venous rings at the level of each vertebra, found most
strikingly at the foramen magnum. Tunneling the bony structure of each vertebral
body is the basivertebral vein, which has a small valve-like opening as it
joins the anterior internal venous plexus.
13.4.6.7 The intervertebral veins
leave the spinal cord through the intervertebral foramina in company with the
spinal nerves. They also have a valve-like mechanism as they join the
intercostal, lumbar and sacral veins.
13.4.6.8
The veins of the spinal cord are minute and delicate. They emerge from
the anterior median fissure as the anterior central vein, and from the
posterior sulcus as the posterior central vein. There are also two lateral
longitudinal veins on either side of the spinal cord, and they all empty into
the intervertebral veins. How- ever, those near the foramen magnum empty into
the inferior petrosal sinus or cerebellar veins.
13.5 Exit of Spinal Nerves Ciba 50
13.5.1
As the anterior root of the spinal cord emerges from the anterior and
lateral gray columns, it traverses the surrounding membranes of pia, arachnoid
and dura. The posterior root, which is attached to the posterolateral portion
of the spinal cord, originates from two bundles of fibers in the spinal
ganglion. Both anterior and posterior roots pierce the dura separately as they
make their exit through their respective intervertebral foramina. As a rule,
the posterior root is thicker and larger than the anterior root. They are
enclosed in a common dural sheath just beyond the spinal ganglion, where they
become the spinal nerve and are surrounded by epineurium.
13.5.2
The spinal ganglia, which lie at the outer portion of the intervertebral
foramina, are oval-shaped and vary in size corresponding to their nerve roots.
13.5.3
The Spinal nerves lie horizontally in the cervical region but below these
segments the spinal nerves assume an increasingly oblique and downward
direction as they approach the lumbar region where they are almost vertical,
forming the cauda equina. At the lower thoracic level, there is a
difference of two vertebral segments between the origin of the spinal nerve and
the level of exit. From each sympathetic trunk ganglion, which lies on the
posterolateral surface of the vertebral body, a branch (gray ramus communicans)
joins the adjacent spinal nerve.
13.5.4
Efferent, preganglionic sympathetic fibers (white ramus communicans),
which originate in the lateral column, pass along with the anterior root to the
corresponding sympathetic ganglion or along its trunk to sympathetic plexuses.
13.5.5
Shortly after emerging from the intervertebral foramen each spinal nerve
gives off a meningeal branch, which turns back through the same foramen to
supply the spinal cord membranes, blood vessels, intervertebral ligaments and
joint surfaces.
13.5.6
The spinal nerve then divides into two branches, each with fibers from
both roots: I. Anterior division supplies the anterior and lateral portions of
the trunk and limbs.
13.5.7
In the thoracic region it spans the space between the pleura and
intercostal membrane, runs below the lower rib margin and supplies the
intercostal muscles and adjacent skin.
13.5.8
In the cervical and lumbar regions the anterior divisions form plexuses.
2. Posterior division is directed backward shortly beyond the formation of the
spinal nerve. Its medial branch Supplies the multifides, longissimus,
semispinalis and trapezius, then proceeds along the spinous process, and
supplies the skin. Its lateral branch traverses the longissimus and supplies
the intercostal muscle and adjacent skin.
13.5.9
In the lumbar region the medial branches of the posterior division hug
the articular processes of the vertebrae and end in the multifides, and the
lateral branches supply the group of sacrospinalis muscles, adjacent fascia and
skill.
13.6
Posterior aspect of the cervical segment of the dura mater (right
half) Photo At 69
13.6.1
To demonstrate the deep structures contained within the vertebral
column) the following steps are carried out:
13.6.2
All muscles from the back of neck are removed
13.6.3
The vertebra/laminae) together with the spinous processes) are
removed.
13.6.4
The content of the epidural space (connective tissue and venous
plexus) is removed from the posterior aspect of the dura mater
13.6.5
The intervertebral foramina are enlarged posteriorly
13.6.6
Several vertebrarterial foramina are opened posteriorly.
13.6.7
Following such a dissection the extensions of the dura mater to
the spinal ganglia, distribution of spinal nerves into the rami,
grouping of the ventral rami, and the relationship of the vertebral
artery to the transverse processes and the spinal nerves become
'apparent.
13.7.1
Nerves are such delicate things if you
look at them laid bare by the scalpel. A spider web latticework connects our brain
to the rest of our body. When the connection is broken, we loose sensation and
function. When nerves are compressed or irritated by our own body’s chemicals
we ache and moan. They cause the muscles to bunch up tight into a knot, which
begs for release.
13.7.2
There are two basic kinds of nerves,
sensory and motor. Sensory nerves
supply the body sensation and motor nerves innervate the muscles and allow you
to move. The structure of these nerves
and the way they exit the spinal cord and supply the rest of the body with
sensation and movement is significant because they pass through structures,
which can irritate and cause pain. In
addition, depending on which part of the nerve is irritated may determine where
the pain is felt.
13.7.3
The
spinal roots exit the spinal cord as either sensory (posterior root) or
motor (anterior root). Each dorsal root has a swelling (ganglion)
outside the spinal cord. The ganglion
contains the cell bodies (neurons) whose dendrites transmit stimulation from
the periphery. The axon continues from
the ganglion to the spinal cord on its way to the brain. Motor nerves contain
axons and sensory nerves consist of dendrites. The spinal nerves combine and
exit the spine via the intervertebral foramen. Shortly after exiting the spine
these combined sensory and motor nerves form the anterior ramus,
posterior ramus) and meningeal branches. The anterior and posterior rami both contain sensory and motor fibers.
13.7.4
The
posterior ramus innervates the muscles of the back, branches into the cutaneous
nerves that supply the skin of the back, and branch into the dermatomes. The
dermatomes and cutaneous nerves overlap each other in supplying the skin with
sensitivity, more or less supplying the same areas at each spinal level.
13.7.5
The
anterior rami of the spinal nerve innervate the muscles, joints, and skin of
the extremities. the anterior ramus also supplies cutaneous sensitivity to the
lateral and anterior trunk.
13.7.6
The
meningeal branch re-enters the spinal canal through the intervertebral foramen
and supplies the vertebrae, vertebral ligaments, blood vessels of the spinal
cord, and the meninges.
13.7.7
13.7.8
Spinal nerves are the paths of communication
between the brain, spinal cord tracts and the periphery.
13.7.9
Each pair of spinal nerves is connected to a
segment of the cord by two points of attachment called roots.
13.7.10 The
posterior dorsal (sensory) root contains sensory nerve fibers only and
conducts nerve impulses from the periphery to the spinal cord.
13.7.11 Each
dorsal root also has a swelling the posterior or dorsal (sensory) root
ganglion, which contains the cell bodies of the sensory neurons from the
periphery.
13.7.12 The
other point of attachment of the spinal nerve to the cord is the anterior or
ventral (motor) root. It contains motor neuron axons only and conducts
impulses from the spinal cord to the periphery.
13.7.13 These
two roots motor and sensory form one nerve, which then exits the spine via the
intervertebral foramen.
13.7.14 Shortly
after a spinal nerve leaves its intervertebral foramen, it divides into several
branches. These branches are known as rami (RA-mi).
13.7.15 The
dorsal ramus (Ramus) innervates the deep muscles and skin of the dorsal
surface of the back.
13.7.16 The
ventral ramus of a spinal nerve innervates the muscles and structures of
the extremities and the lateral and ventral trunk.
13.7.17 In
addition to dorsal and ventral rami, spinal nerves also give off a meningeal
branch.
13.7.18
This branch reenters the spinal canal through
the intervertebral foramen and supplies the vertebrae, vertebral ligaments,
blood vessels of the spinal cord, and the meninges.
13.7.19
13.8
Disorders:
Homeostatic Imbalances
13.9
The
Brain and Cranial Nerves
13.10
The
Sensory, Motor and Integrative Systems
13.11
The
Autonomic Nervous System
13.12
The
Special Senses
14.1 Principal Parts
14.1.1
The brain is mushroom shaped, weighs
about 3 lbs., is composed of 1,000,000,000,000 (1 trillion) neurons and as such
is one of the largest organs in the body.[6] It is divided into four parts the brain stem, diencephalon,
cerebrum, and cerebellum. The brain
stem, which is the stock of the mushroom, consists of the medulla oblongata,
pons, and midbrain. Superior to brain
stem is a diencephalon which contains the thalamus and hypothalamus. The cerebrum accounts for most of the weight
of the brain (7/8th) and occupies most of the cranium. Underneath this cerebrum and behind the
brainstem is the cerebellum.
14.2 Protection and Coverings
14.2.1
The brain has the same protective
coverings as the spinal cord e.g. dura mater, arachnoid, and pia mater. The
cranial dura mater consists of two layers.
The outer layer is thicker and adheres tightly to the cranial bones.
This outer layer is not found covering the spinal cord. The dura maters second meningeal layer is
contiguous with the meningeal layer of the spinal cord.
14.3 Cerebrospinal fluid and ventricles
14.3.1
There are four ventricles, two of
which are called the lateral ventricles.
The lateral ventricles are on either side of the right and left halves
of the brain. These two ventricles are
connected on both sides by the interventricular foramen to the third ventricle. The third ventricle is a vertical slit
between both halves of the thalamus an between the two lateral ventricles. The fourth ventricle is connected by the
cerebral aqueduct to the third ventricle.
The fourth ventricle is connected to the sub arachnoid space via the two
lateral apertures and to the central canal via median aperture. Contained in the ventricles, the central
canal, and sub-arachnoid space is 3-5 oz. of cerebrospinal fluid. The cerebrospinal fluid is composed of
proteins, glucose, urea, lymphocytes, and salts. Cerebrospinal fluid is
exchanged between capillaries and ependymal cells, which form plexuses along
the roof of all of the ventricles. The
lining in these areas of the ventricles is composed of ependymal cells
(specialized neuroglial cells that form the lining of all of the ventricles)
and capillaries, which form these plexuses called choroid plexuses. These choroid plexuses allow exchange of
proteins, glucose, urea, lymphocytes, and salts between the bloodstream and
ventricles. The byproducts of the
metabolism of brain cells are filtered into the bloodstream for removal and
nutrients necessary for healthy brain cells are exchanged from the bloodstream
to the ventricles. the quarry plexuses
form and effective Lund cerebrospinal fluid barrier that prevents coccyx up to
30 from entering the ventricles but.
Can nutrients. The blood brain
barrier or blood cerebrospinal fluid barrier he's a protective mechanism in
each ventricles to prevent substances from harming midbrain. One possible schematic for the circulation
of cerebrospinal fluid (CSF) in the brain is as follows; CSF is produced by the
choroid plexuses from the lateral ventricles, which then circulates through the
interventricular foramen to the third ventricle. In the 3rd ventricle, more CSF is added by the choroid plexuses
flowing to the 4th ventricles via the cerebral Aqueduct. At the 4th ventricles more C S F is added
from the choroid plexuses and then continues on to the sub arachnoid space via
the lateral aperture where it flows around the back of the brain and posterior
spinal cord. Cerebrospinal fluid also
can flow through the median aperture both to the sub arachnoid space around the
anterior portion of brain and the sub arachnoid space around the anterior portion
of the spinal cord. From the median
aperture, CSF can flow down the central canal of the spinal cord. Cerebrospinal
fluid is gradually absorbed into the veins after circulating through the
ventricles. Additional cerebrospinal
fluid can also be formed by ependymal (neuroglial) cells in a central canal the
spinal cord. This flows up the central
canal to be added to the full in the 4th ventricles. Cerebrospinal fluid is absorbed into the veins via the arachnoid
using finger like projections called arachnoid villi. These finger like projections push through dural venous sinuses
such as the superior sagittal sinus.
CSF is absorbed as fast as it is produced.
14.3.2
When CSF is prevented from flowing
because of an obstruction in the ventricles for in the sub arachnoid space it
is called hydrocephalus. Internal
hydrocephalus is caused from a blockage in the ventricles and external
hydrocephalus is caused by blockage of the arachnoid space.
14.3.3
One principal function of the cerebrospinal
fluid are to serve as a shock-absorbing medium to protect the brain &
spinal cord from injury, which would otherwise cause them to crash against the
bony walls of the cranium and vertebral canal.
The second principal function of the cerebrospinal fluid is to deliver
nutrition to the brain cells and remove waste and toxic substances.
14.4
Blood Circulation
14.4.1
The cerebrospinal circle (Circle of
Willis) supplies the brain with oxygen and nutrients. The blood vessels that pass along the outer surface of the brain
and penetrate inward are surrounded by pia mater. The space between the pia mater and the penetrating blood vessel
is called perivascular space. The brain
uses 20% of the oxygen supply utilized by the entire body yet represents only
2% of its weight. The brain is very
active metabolically and can only tolerate short periods without the necessary
supply of oxygen. Four minutes of
oxygen, deprivation may result in permanently injured brain cells. Additionally the lysosomes in brain cells
release enzymes, which bring about the self-destruction of the cell. Babies deprived of oxygen while going thru
the birth canal can suffer mental retardation, epilepsy, and or paralysis. Because glucose is the primary fuel of the
brain cells deprivation can result in mental confusion, dizziness, convulsions
and unconsciousness. -
14.4.2
When the brain is the deprived of
oxygen, there is an increase in carbon dioxide (CO2 O2). Both deprivation of oxygen and increase in carbon
dioxide can create can increase asset dilation of the blood vessels to the
brain. Carbon dioxide does this by
combining with water to form carbonic acid (H2CO3). Carbonic acid breaks down further into
hydrogen ions (H+) bicarbonate ions (HCO3). The hydrogen
ions (H+) increased vasodilatation of the cerebral vessels.
14.4.3
The blood brain barrier (BBB) allows
certain substances such as glucose and oxygen to rapidly pass from the
capillaries into the brain cells.
Capillaries of the brain are constructed differently than other
capillaries in the body. Their cells
and more densely packed and large numbers of astrocytes (a type of neuroglial
cell) are also present. These astrocytes
produce substance, which influence the capillaries and increase or decrease their
permeability.
14.5
Brain Stem
14.5.1
Medulla Oblongata (Medulla)
14.5.1.1
The medulla is about an inch long and
lies just above the foramen magnum. As
such, it is a continuation the spinal cord and serves as a relay station for
all nerve tracks passing to and from the spinal cord. Some of these ascending and he descending nerve tracks cross to
the opposite side as they pass through medulla. The largest of these nerve tracks pass on the anterior side of
the medulla and form pyramids that cross to the opposite side and are called
the decussation of the pyramids. This
is why motor movements of one side of the body are controlled by the opposite
side of the brain. There is no known
adaptive value for these phenomena.
There is a region of gray matter in the medulla called the reticular
formation. The reticular formation is
also located in the spinal cord, Pons, midbrain and diencephalon. The reticular formation connects to many
parts of the brain and is involved in arousal from sleep. The medulla also contains some of the
cranium nerves.
14.5.1.2
The vestibulocochlear (VIII) nerve is
concerned with hearing and equilibrium; the glossopharyngeal (IX) nerve is
concerned with swallowing, salvation, and taste; the vagus (X) relays nerve
impulses to from many of the thoracic and abdominal viscera; the cranial
portion of the accessory (XI) nerve originates in the 5 cervical vertebrae of
the spinal cord. The accessory (XI)
nerve conveys nerve impulses related to head and shoulder movements. The glossopharyngeal (IX) convey nerve impulses
that involve tongue movements.
14.5.1.4
There are three reflex centers in the
medulla; the cardiac center, which regulates the rate of heart, beat the force
of contraction; the medullary rhythmicity area, which adjusts the basic rhythm
of breathing; and the vasoconstrictor center, which regulates the diameter of
blood vessels. The medulla also has
centers, which control following, vomiting, coughing, sneezing, and hiccuping.
14.5.2
Pons
14.5.2.1
The Pons (Bridge) is about an inch
long and as the name implies connects the spinal cord with the brain and parts
of the brain with each other. It lies
directly above the medulla and is anterior to the cerebellum. The connections it makes in the brain are in
two principal directions e.g. the transverse fibers connect with the cerebellum
and the longitudinal fibers of the Pons belong to the motor and sensory tracks
that connect the spinal cord for medulla with the other parts of the brain stem.
14.5.2.2
The following cranial nerves have
neurons, which originate in the Pons. The trigeminal (V) nerve controls
impulses for chewing and for sensations of the head and face. The abducens (VI)
nerve regulates certain, eyeball movements. The facial (VII) nerve, which
conducts impulses, related to taste, salvation, and facial expression. The
vestibulocochlear (VIII) nerve (vestibular branches), is concerned with
equilibrium.
14.5.2.3
The pneumotaxic area and apneustic
area together with the medullary rhythmicity area in the medulla control
respiration (breathing movements).
14.5.2.4
Oculomotor (III) trochlear (IV)
lemniscus mesencephalon that the tectum
corpora quadrigemina colliculi
substanti nigra
14.5.3
Midbrain
14.5.3.1
The midbrain extends from the pons to
the lower portion of the diencephalon.
14.6
Diencephalon
14.6.1
The diencephalon consists principally
of this thalamus and hypothalamus.
14.6.2
Thalamus
14.6.2.1
This thalamus is an oval structure
above the midbrain that measures about 1 in. in length and constitutes 4/5 of
the diencephalon.
14.6.3
Test your Knowledge
Click
the link below to take the section test.
Section 2.2.1 Test 2
14.7
Cerebrum
14.7.1
Lobes
14.7.2
White
Matter
14.7.3
Basal
Ganglia (Cerebral Nuclei)
14.7.4
Limbic
System
14.7.5
Functional
Areas of Cerebral Cortex
14.7.6
Electroencephalogram
(EEG)
14.8
Brain
Lateralization (Split-Brain Concept)
14.9
Cerebellum
14.9.1
Structure
14.9.2
Functions
14.10
Neurotransmitters
in the Brain
14.10.1
Neurotransmission
Merck
14.10.1.1 General
14.10.1.1.1
A
nerve cell (neuron) has two major functions: propagation of an action potential
(nerve impulse, signal) along its axon and transmission of this signal from one
neuron to another neuron or to an effector cell to elicit a response. Effector
cells include skeletal and cardiac muscles and exocrine and endocrine cells
regulated by the nervous system. Impulse conduction along an axon is
electrical, caused by the exchanges of Na+ and K+ ions
across the neuronal membrane. In contrast, impulse transmission from one neuron
to another neuron or to a non-neuronal effector cell depends on the action of
specific neurotransmitters on specific receptors.
14.10.1.1.2
A
particular neuron generates an identical action potential after each stimulus
and conducts it at a fixed velocity along the axon. Velocity depends on axonal
diameter and degree of myelination. For myelinated fibers, velocity (m/sec) is
approximately 3.7 times the diameter (µ); eg, for a large (20-µ) myelinated
fiber, velocity is nearly 75 m/sec. For unmyelinated fibers with diameters of 1
to 4 µ, velocity is 1 to 4 m/sec.
14.10.1.1.3
A
neuron simultaneously receives many stimuli--positive and negative--from other
neurons and integrates them into various patterns of firing. The nerve impulses
travel down axons to the next synapse. Once axonal propagation begins, drugs or
toxins can modify the amount of neurotransmitter released from the terminal
axon. For example, botulinum toxin blocks release of acetylcholine. Other
chemical factors can also influence the effect of transmission by modifying a
receptor. In myasthenia gravis, antibodies block the nicotinic acetylcholine
receptor.
14.10.1.1.4
Synapses
occur between neuron and neuron and, in the periphery, between neuron and
effector (eg, a muscle); in the CNS, more complex arrangements exist.
Functional contact between two neurons may occur between axon and cell body,
axon and dendrite (the receiving area of a neuron), cell body and cell body, or
dendrite and dendrite. Neurotransmission can increase or decrease to generate a
physiologic function or to respond to changing physiologic needs. Many
neurologic and psychiatric diseases are caused by pathologic overactivity or underactivity
of neurotransmission. Many drugs can modify neurotransmission; some (eg,
hallucinogens) cause adverse effects, and some (eg, antipsychotic drugs)
correct pathologic conditions.
14.10.1.1.5
Development
and maintenance of cells in the nervous system depend on many specific
proteins, such as nerve growth factor, brain-derived neurotrophic factor, and
neurotrophin-3.
14.10.1.2 Basic Principles of Neurotransmission
14.10.1.2.1
The
nerve cell body produces enzymes that are involved in the synthesis of most
neurotransmitters. The enzymes act on precursor molecules taken up by the
neuron to form the neurotransmitter. The neurotransmitter is stored at the
nerve terminal in vesicles (see Fig. 166-1). The amount in one vesicle (usually several thousand
molecules) is a quantum. Some neurotransmitter molecules are constantly
extruded from the terminal, but the amount is insufficient to elicit a
significant physiologic response. An action potential arriving at the terminal can
activate calcium currents and precipitate the simultaneous release of
neurotransmitter molecules from many vesicles by fusing the membrane of the
vesicles to that of the nerve terminal. An opening forms through which the
molecules are expelled into the synaptic cleft by exocytosis.
14.10.1.2.2
The
quantity of neurotransmitters in the terminals is kept relatively constant and
independent of nerve activity by tight regulation of neurotransmitter
formation. Regulation varies among neurons and is achieved by modifying precursor
uptake or the activity of enzymes involved in neurotransmitter synthesis or
destruction. Stimulation or blockade of postsynaptic receptors can decrease or
increase presynaptic neurotransmitter synthesis.
14.10.1.2.3
Neurotransmitters
diffuse across the synaptic cleft, bind briefly to receptors, and activate
them, causing physiologic responses. Depending on the receptor, the response
may be excitatory (ie, initiating a new action potential) or inhibitory (ie,
inhibiting the development of a new action potential).
14.10.1.2.4
The
neurotransmitter-receptor interaction must be terminated quickly so that the
same receptors can be rapidly activated repeatedly. The neurotransmitter is
quickly pumped back into the presynaptic nerve terminals by active processes
(reuptake), is destroyed by enzymes near the receptors, or diffuses into the
surrounding area and is destroyed.
14.10.1.2.5
Abnormalities
of neurotransmitter synthesis, storage, release, or degradation or changes in
the number and affinity of receptors can affect neurotransmission and cause
clinical disorders (see Table 166-1).
14.10.1.3 Major Neurotransmitters
14.10.1.3.1
A
neurotransmitter is a chemical that is selectively released from a nerve
terminal by an action potential, interacts with a specific receptor on an
adjacent structure, and, if received in adequate amounts, elicits a specific
physiologic response. To be a neurotransmitter, a chemical must be present in
the nerve terminal, be released from the nerve terminal by an action potential,
and, when applied experimentally to the receptor, always produce the identical
effect. Many chemicals act as neurotransmitters. There are at least 18 major
neurotransmitters; several occur in slightly different forms.
14.10.1.3.2
The amino
acids glutamate and aspartate are the major excitatory
neurotransmitters in the CNS. They occur in the cortex, cerebellum, and spinal
cord.
14.10.1.3.3
Aminobutyric
acid (GABA)
14.10.1.3.3.1
major
inhibitory neurotransmitter in the brain. GABA is derived from glutamic acid,
which is decarboxylated by glutamate decarboxylase. After interaction with its
receptors, GABA is actively pumped back into the nerve terminals and
metabolized. Glycine, which resembles GABA in its action,
occurs principally in the interneurons of the spinal cord. Glycine is probably
metabolized from serine.
14.10.1.3.4
Serotonin
(5-hydroxytryptamine, or 5-HT)
14.10.1.3.4.1
generated by
the raphe nucleus and midline neurons of the pons and upper brain stem.
Tryptophan is hydroxylated by tryptophan hydroxylase to 5-hydroxytryptophan,
then decarboxylated to serotonin. Serotonin levels are controlled by the uptake
of tryptophan and intraneuronal monoamine oxidase.
14.10.1.3.5
Acetylcholine
14.10.1.3.5.1
major
neurotransmitter of the bulbospinal motor neurons, autonomic preganglionic
fibers, postganglionic cholinergic (parasympathetic) fibers, and many neurons
in the CNS (eg, basal ganglia, motor cortex). It is synthesized from choline
and mitochondrially derived acetyl coenzyme A by choline acetyltransferase.
When released, acetylcholine stimulates specific cholinergic receptors, an
interaction rapidly terminated by local hydrolysis of acetylcholine to choline
and acetate by acetylcholinesterase. Acetylcholine levels are regulated by
choline acetyltransferase and by choline uptake.
14.10.1.3.6
Dopamine
14.10.1.3.6.1
neurotransmitter
of some peripheral nerve fibers and many central neurons (eg, in the substantia
nigra, midbrain, ventral tegmental area, and hypothalamus). The amino acid
tyrosine is taken up by dopaminergic neurons and converted by tyrosine
hydroxylase to 3,4-dihydroxyphenylalanine (dopa), which is decarboxylated by
aromatic-L-amino-acid decarboxylase to dopamine. After release, dopamine
interacts with dopaminergic receptors, and its residue is actively pumped back
(reuptake) into the prejunctional neurons. Tyrosine hydroxylase and monoamine
oxidase regulate dopamine levels in nerve terminals.
14.10.1.3.7
Norepinephrine
14.10.1.3.7.1
neurotransmitter
of most postganglionic sympathetic fibers and many central neurons (eg, in the
locus caeruleus and hypothalamus). The precursor tyrosine is converted to
dopamine, which is hydroxylated by dopamine
-hydroxylase
to norepinephrine. When released, norepinephrine interacts with adrenergic
receptors, a process terminated by its reuptake into the prejunctional neurons
with subsequent degradation by monoamine oxidase and by the action of catechol O-methyltransferase (COMT), which is
located mainly extraneuronally. Tyrosine hydroxylase and monoamine oxidase
regulate intraneuronal norepinephrine levels.
14.10.1.3.8
Endorphin
14.10.1.3.8.1
and other
endorphins are polypeptides that activate many central neurons (eg, in the
hypothalamus, amygdala, thalamus, and locus caeruleus). The cell body contains
a large polypeptide called pro-opiomelanocortin, the precursor of several
neuropeptides (eg,
-,
-,
and
-endorphins).
This polypeptide is transported down the axon and is cleaved into specific
fragments; one is
-endorphin,
which contains 31 amino acids. After release and interaction with opioid
receptors,
-endorphin
14.10.1.3.9
Met-enkephalin and leu-enkephalin
14.10.1.3.9.1
small peptides
present in many central neurons (eg, in the globus pallidus, thalamus, caudate,
and central gray matter). Their precursor, proenkephalin, is formed in the cell
body, then split by specific peptidases into smaller peptides. The resulting
fragments include two enkephalins, each composed of five amino acids and
containing either methionine or leucine terminals. After release and
interaction with peptidergic receptors, the enkephalins are hydrolyzed into
smaller, inactive peptides and amino acids, as are dynorphins and substance P.
14.10.1.3.10
Dynorphins
14.10.1.3.10.1 a group of seven peptides with
similar amino acid sequences. They geographically coexist with enkephalins. Substance
P, a peptide,
occurs in central neurons (habenula, substantia nigra, basal ganglia, medulla,
and hypothalamus) and in high concentration in the dorsal root ganglia. It is
released by intense afferent painful stimuli.
14.10.1.3.10.1.1 Neurotransmitters whose roles in
neurotransmission have been less firmly established include histamine,
vasopressin, vasoactive intestinal peptide, carnosine, bradykinin,
cholecystokinin, bombesin, somatostatin, corticotropin releasing factor,
neurotensin, and possibly adenosine.
14.10.1.4 Major Receptors
14.10.1.4.1
Neurotransmitter
receptors are protein complexes that span the cell membrane. Receptors coupled
to a second messenger are usually monomeric and consist of three parts: the
extracellular part, where glycosylation occurs; the transmembrane part, which
forms a pocket where the neurotransmitter is presumed to act; and the
intracytoplasmic part, where G-protein binding or regulation by phosphorylation
of the receptor occurs. Ion channel receptors are multimeric. In some cases,
activation of the receptor results in modification of channel ion permeability.
In others, activation of a second messenger results in changes of channel
conductance.
14.10.1.4.1.1
Receptors
that are continuously stimulated by neurotransmitters or drugs (agonists)
become hyposensitive (down-regulated); those that are not stimulated by their
neurotransmitter or are blocked chronically by drugs (antagonists) become
hypersensitive (up-regulated). Up- or down-regulation of receptors strongly
influences the development of tolerance and physical dependence. Withdrawal is
usually a rebound phenomenon due to altered receptor affinity or density. These
concepts are particularly important in organ or tissue transplants, in which
the receptors are deprived of the physiologic neurotransmitter by denervation.
14.10.1.4.1.1.1
Most
neurotransmitters interact primarily with postsynaptic receptors, but some
receptors are located on presynaptic neurons, providing fine control of
neurotransmitter release.
14.10.1.4.2
Cholinergic receptors
classified
as nicotinic N1 (in the adrenal medulla and autonomic ganglia) or N2
(in skeletal muscle) or muscarinic M1 (in the autonomic nervous system,
striatum, cortex, and hippocampus) or M2 (in the autonomic nervous
system, heart, intestinal smooth muscle, hindbrain, and cerebellum).
14.10.1.4.3
Adrenergic receptors
classified
as
1
(postsynaptic in the sympathetic system),
2
(presynaptic in the sympathetic system and postsynaptic in the brain),
1
(in the heart), or
2
(in other sympathetically innervated structures). Dopaminergic
receptors are classified as D1, D2, D3,
D4, and D5. D3 and D4 play a role
in thought control (limit negative symptoms of schizophrenic processes),
whereas D2 receptor activation controls the extrapyramidal system.
14.10.1.4.4
GABA receptors
classified
as GABAA (activating chloride channels) and GABAB
(potentiating cAMP formation). The GABAA receptor consists of several
distinct polypeptides and is the site of action for several neuroactive drugs,
including benzodiazepines, newer anticonvulsants (eg, lamotrigine),
barbiturates, picrotoxin, and muscimol.
14.10.1.4.5
Serotoninergic (5-HT) receptors
(with
at least 15 subtypes) are classified as 5-HT1 (with four subtypes),
5-HT2, and 5-HT3. 5-HT1A receptors, which
occur presynaptically in the raphe nucleus (inhibiting presynaptic uptake of
5-HT) and postsynaptically in the hippocampus, modulate adenylate cyclase. 5-HT2
receptors, located in the fourth layer of the cortex, are involved in
phosphoinositide hydrolysis (see Table 166-2). 5-HT3
receptors occur presynaptically in the nucleus tractus solitarius.
14.10.1.4.6
Glutamate receptors
classified
as ionotrophic NMDA (N-methyl-D-aspartate) receptors, which bind NMDA,
glycine, zinc, Mg++, and phencyclidine (PCP, also known as angel
dust) and affect the influx of Na+, K+, Ca++,
and non-NMDA receptors, which bind quisqualate and kainate. Non-NMDA channels
are permeable to Na+ and K+ but not to Ca++.
These excitatory receptors mediate important toxic effects by increasing
calcium, free radicals, and proteinase. In neurons, synthesis of nitric oxide
(NO) involving NO synthase increases in response to glutamate.
14.10.1.4.7
Endorphin-enkephalin (opioid) receptors
classified
as µ1 and µ2 (affecting sensorimotor integration and
analgesia),
1
and
2
(affecting motor integration, cognitive function, and analgesia), and
1,
2,
and
3
(affecting water balance regulation, analgesia, and food intake). Sigma
receptors, currently classified as nonopioid and mostly localized in the
hippocampus, bind PCP.
14.10.1.5 Neurotransmitter Transport
14.10.1.5.1
Two types of
neurotransmitter transporters are essential for neurotransmission. The uptake
carrier, located on presynaptic neurons and plasma cells, pumps neurotransmitters
from the extracellular space into the cell. It replenishes the neurotransmitter
supply, helps terminate the action of the neurotransmitter, and, in the case of
glutamate, keeps the amount of neurotransmitter below toxic levels. The energy
required by these pumps is provided by ATP. The other type of transporter,
located on the membrane of the vesicle, concentrates the neurotransmitter into
the vesicles for further exocytosis. These transporters are powered by
cytoplasmic pH and the voltage gradient across the vesicular membrane. During
anoxia or ischemia, the transmembrane ionic gradient changes, and glutamate is
transported from the vesicles into the cytoplasm, increasing the intracellular
concentration of glutamate to potentially lethal levels.
14.10.1.5.2
Second messenger systems
14.10.1.5.2.1
consist of
regulatory G proteins and catalytic proteins (eg, adenylate cyclase,
phospholipase C) that link receptors and effectors. A second messenger can be
the trigger of a chain reaction or the target of a regulatory pathway (eg,
calcium--see Table 166-2).
14.11
Cranial
Nerves
14.12
Aging
and the Nervous System
14.13
Developmental
Anatomy of the Nervous System
18.1
Endocrine
Glands
18.2
Chemistry
of Hormones
18.3
Physiology
of Hormonal Action
18.3.1
Overview
18.3.2
Receptors
and Hormone Specificity
18.3.3
Interaction
with Plasma Membrane Receptors
18.3.4
Interaction
with Intracellular Receptors
18.3.5
Hormonal
Interactions
18.3.6
Prostaglandins
(PGs) and Hormones
18.4
Control
of Hormonal Secretions: Feedback Control
18.5
Pituitary
(Hypophysis)
18.5.1
Adenohypophysis
18.5.1.1
Human
Growth Hormone (hGH)
18.5.1.2
Thyroid
Stimulating Hormone (TSH)
18.5.1.3
Adrenocorticotropic
Hormone (ACTH)
18.5.1.4
Follicle
Stimulating Hormone (PSH)
18.5.1.5
Luteinizing
Hormone (LH)
18.5.1.6
Prolactin
(PRL)
18.5.1.7
Melanocyte
Stimulating Hormone (MSH)
18.5.2
Neurohypophysis
18.5.2.1
Oxytocin
(OT)
18.5.2.2
Antidiuretic
Hormone (ADH)
18.6
Thyroid
18.6.1
Formation,
Storage, and Release of Thyroid Hormones
18.6.2
Function
and Control of Thyroid Hormones
18.6.3
Calcitonin
(CT)
18.7
Parathyroids
18.7.1
Parathyroid
Hormone (PTH)
18.8
Adrenals
(Suprarenals)
18.8.1
Adrenal
Cortex
18.8.1.1
Mineralocorticoids
18.8.1.2
Glucocorticoids
18.8.1.3
Gonadocorticoids
18.8.2
Adrenal
Medulla
18.8.2.1
Epinephrine
and Horepinephrine (NE)
18.9
Pancreas
18.9.1
Glucagon
18.9.2
Insulin
18.10
Ovaries
and Testes
18.11
Pineal
(Epiphusis Cerebrl)
18.12
Thymus
18.13
Aging
and the Endocrine System
18.14
Developmental
Anatomy of the Endocrine System
18.15
Other
Endocrine Tissues
18.16
Growth
Factors
18.17
Stress
and the General Adaptation Syndrome
18.17.1
Stressors
18.17.2
Alarm
Reaction
18.17.3
Resistance
Reaction
18.17.4
Exhaustion
18.17.5
Stress
and Disease
19.1
Blood
19.1.1
Physical
Characteristics of Blood
19.1.2
Functions
of Blood
19.1.3
Components
of Blood
19.1.3.1
Erthrocytes
19.1.3.2
Leucocytes
19.1.3.3
Thrombocytes
19.1.3.4
Plasma
19.1.4
Physiology
of Hemostosis
19.1.5
Grouping
(Typing) of Blood
19.1.6
Interstitial
Fluid and Lymph
19.1.7
Disorders:
Homeostatic Imbalances
20.1
Location
of Heart
20.2
Pericardium
20.3
Wall;
Chambers; Vessels; and Valves
20.4
Blood
Supply
20.5
Conduction
System
20.6
Electrocardiogram
(ECG)
20.7
Blood
Flow through the Heart
20.8
Physiology
of Cardiac Cycle
20.9
Physiology
of Cardiac Output (CO)
20.10
Artificial
Heart
20.11
Diagnosing
Heart Disorders
20.12
Heart-Lung
Machine
20.13
Risk
Factors in Heart Disease
20.14
Developmental
Anatomy of the Heart
20.15
Disorders:
Homeostatic Imbalances
21.1
Arteries
21.2
Arterioles
21.3
Capillaries
21.4
Venules
21.5
Veins
21.6
Blood
Reservoirs
21.7
Physiology
of Circulation
21.8
Shock
and Homeostasis
21.9
Checking
Circulation
21.9.1
Pulse
21.9.2
Measurement
of Blood Pressure
21.10
Circulatory
Routes
21.10.1
Systemic
Circulation
21.10.1.1
Arteries
of Pelvis and Lower Extremities
21.10.1.1.1
External
Iliacs
21.10.1.1.1.1
External Iliac arteries diverge through greater (false)
pelvis, enter thighs, and here become right and left femoral arteries.
21.10.1.1.1.2
Both femorals send branches back up to genitals and wall of
abdomen.
21.10.1.1.1.3
Other branches run to muscles of thigh.
21.10.1.1.1.4
Femoral continues down medial and posterior side of thigh at back
of knee joint, where it becomes the popliteal artery.
21.10.1.1.1.5
Between knee and ankle, popliteal runs down back of leg and is
called posterior tibial artery.
21.10.1.1.1.6
Below knee, peroneal artery branches off posterior tibial to
supply structures on medial side of fibula and calcaneus.
21.10.1.1.1.7
In calf, anterior tibial artery branches off popliteal and
runs along front of leg.
21.10.1.1.1.8
At ankle, it becomes dorsalis pedis artery.
21.10.1.1.1.9
At ankle, posterior tibial divides into medial and lateral
plantar arteries.
21.10.1.2
Veins
of Lower Extremities
21.10.1.2.1
Blood from
each lower extremity is returned by superficial set and deep set of veins.
21.10.1.2.2
Superficial
Veins
21.10.1.2.2.1
Great Saphenous
21.10.1.2.2.1.1
This is the longest vein in the body and begins at the medial
end of the dorsal venous arch of foot.
21.10.1.2.2.1.2
It passes in front of medial Malleolus and then upward along
medial aspect of leg and thigh.
21.10.1.2.2.1.3
It receives tributaries from superficial tissues and connects
with deep veins as well.
21.10.1.2.2.1.4
It empties into femoral vein in groin.
21.10.1.2.2.2
Small Saphenous
21.10.1.2.2.2.1
This vein begins at lateral end of dorsal venous arch of foot.
21.10.1.2.2.2.2
It passes behind lateral Malleolus and ascends under skin of
back of leg.
21.10.1.2.2.2.3
It receives blood from foot and posterior portion of leg.
21.10.1.2.2.2.4
It empties into popliteal vein behind knee.
21.10.1.2.3
Deep Veins
21.10.1.2.3.1
Posterior Tibial
21.10.1.2.3.1.1
Union of medial and lateral plantar veins behind medial
Malleolus forms this vein.
21.10.1.2.3.1.2
It ascends deep in muscle at back of leg, receives blood from
peroneal vein, and unites with anterior tibial vein just below knee.
21.10.1.2.3.2
Anterior Tibial
21.10.1.2.3.2.1
This vein is the upward continuation of dorsalis pedis veins
in foot.
21.10.1.2.3.2.2
It runs between tibia and fibula and unites with posterior
tibial to form popliteal vein.
21.10.1.2.3.3
Popliteal
21.10.1.2.3.3.1
This vein is just behind knee, receives blood from anterior
and posterior tibials and small Saphenous vein.
21.10.1.2.3.4
Femoral
21.10.1.2.3.4.1
This vein is the upward continuation of popliteal just above
knee.
21.10.1.2.3.4.2
Femorals run up posterior of thighs and drain deep structures
of thighs.
21.10.1.2.3.4.3
After receiving great Saphenous veins in groin, they continue
as right and left external iliac veins
21.10.2
Pulmonary
Circulation
21.10.3
Hepatic
Portal Circulation
21.10.4
Fetal
Circulation
21.11
Exercise
and the Cardiovascular System
21.12
Aging
and the Cardiovascular System
21.13
Developmental
Anatomy of Blood and Blood Vessels
21.14
Disorders:
Homeostatic Imbalances
22.1